Hadean Earth & Archean Climate
Contents
7. Hadean Earth & Archean Climate#
7.1. Hadean Earth#
The period from the formation of the Earth (\({\sim}4.56\ {\rm Ga}\)) up to the age of the oldest rocks (\({\sim}3.8-4\ {\rm Ga}\)) is referred to as the Hadean eon. The term Hadean refers to the classical Greek domain of Hades, which our modern sensibilities would call hell. The naming is apt because all of the current evidence indicates that the Hadean Earth was very hot and extremely active on the surface, with widespread volcanic activity and frequent impacts. This time encompasses the assemblage of Earth from the smaller planetesimals, dramatic internal rearrangement such as core formation, the creation of the ocean and earliest atmosphere, and the origin of Earth’s Moon. Forces that acted on Earth were essentially the same as those acting on Mars and Venus, and a traveler visiting Earth would have seen little to distinguish it from the two neighboring terrestrial planets.
7.1.1. Internal Structure of Earth#
We cannot drill more than a few kilometers into the solid Earth with current technologies, yet the center of the Earth lies over \(6000\ {\rm km}\) from the surface. Apollo astronauts drilled only a meter into the Moon, and spacecraft have dug even less deeply into Mars. But, the European Space Agency (ESA) will send a rover (in the 2020s) to drill 2 meters into the Martian surface. How can we possibly know anything of the internal structure of these planets if drilling significant distances is not possible?
Mapping the gravitational fields of the terrestrial planets reveals important information akin to that for the giant planets. Such mapping has revealed the root structure of the continents on Earth, information on the patterns of convection in Earth’s mantle, the nature of the structure beneath the highlands on Venus, and the presence of very dense rock under portions of the Moon’s surface.
Earthquakes provide the key to inferring the structure of Earth, which is deduced from a network of seismometers. A limited network of seismometers exist on the Moon from the Apollo landings. A seismometer was part of the scientific instrument panel of the Mars Insight Mission, which detected a magnitude 5 quake. An earthquake is an oscillatory movement of the ground, generated by the sudden slippage of rock along a fault in Earth’s crust.
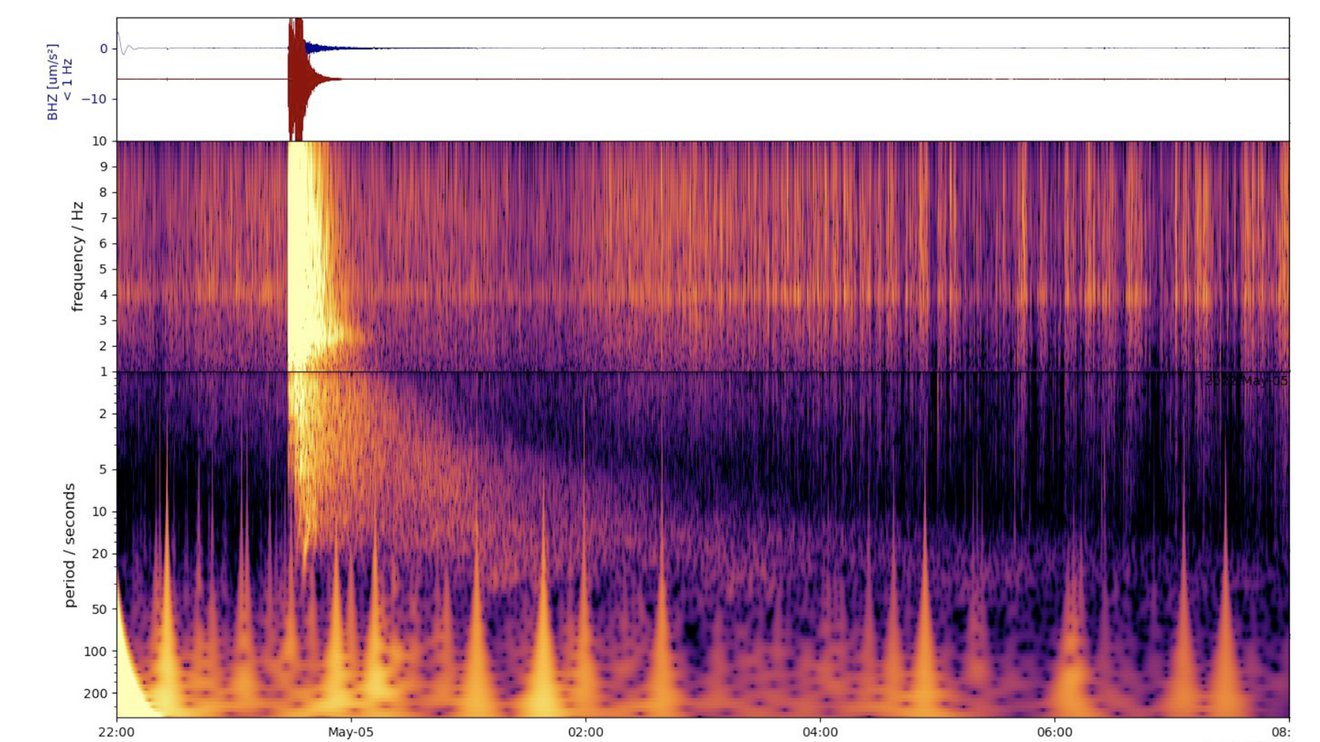
Fig. 7.1 This spectrogram shows the largest quake ever detected on another planet. Estimated at magnitude 5, this quake was discovered by NASA’s InSight lander on May 4, 2022, the 1,222nd Martian day, or sol, of the mission. Credit: NASA/JPL-Caltech/ETH Zurich.#
Earthquake waves travel around and through Earth because solid rock is an excellent medium for conducting the wave motion. Rock motions include compression-rarefaction of the rock (P-waves), and shearing motions through S-waves. P-waves move easily through solid or liquid media, while S-waves cannot move through liquid but instead are damped out because the shear forces cannot be maintained.
Seismometers measure the amplitude of the waves and the time of arrival at various stations from a given earthquake. From this information, the velocity of the seismic waves can be determined, where shifts in velocity correspond to waves encountering different media through reflection and refraction. This also makes it possible to precisely locate the origin point of an earthquake.
Through seismic waves, geologists have determined that the Earth has a distinct core, which is determined through the refraction of P-waves and the shadows of S-waves that cannot penetrate the core. The bending path of P-waves indicates that Earth’s core has a different density and/or composition from the mantle (material above it). The core can be liquid or solid, where the inner core is solid and is surrounded by the liquid outer core. The core is an alloy of nickel and iron (with less than 10% \(\rm Ni\)), where the nickel is required to lower the melting point of iron so that the outer core can exist as a liquid.
Note
It is a common chemical fact that combining certain elements or molecules that are compatible in terms of atomic size or valence can allow substances to liquify or solidify at lower temperatures. Iron combined with oxygen or sulfur forms solutions and yields the right melting properties for the Earth’s central pressure (4 million atmospheres).
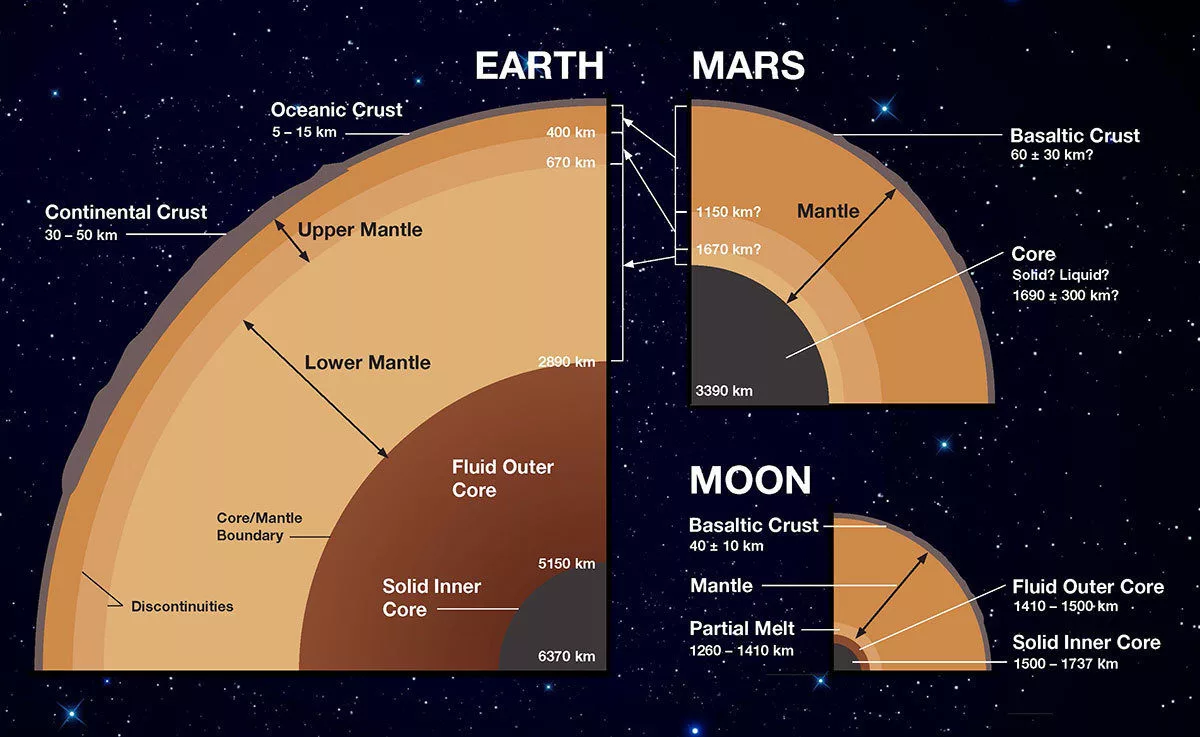
Fig. 7.2 All of the terrestrial planets have a three-part layered structure. At the center is a metallic, iron-rich core, part of which may be molten. Above the core is a thick middle layer called the mantle, made of silicate rock (composed mostly of silicon, oxygen, iron, and magnesium), making up most of the bulk of the planet. Above the mantle is a relatively thin crust of less-dense rocky material. The crust has more lighter elements (aluminum, sodium, calcium, sulfur) than the mantle. Image Credit: NASA / JPL.#
Outside the core there is structure in terms of chemical composition and physical density changes. The chemical change is from crustal rocks that are rich in silicon and aluminum to mantle rocks rich in silicon and magnesium.
Changes in the seismic velocity are seen in the upper \(700\ {\rm km}\) of the Earth’s mantle, which indicate transitions from lithosphere (stiff upper layer) and the asthenosphere (soft, plastic layer).
At \(400\ \rm km\), there is a sharp change in velocity due to the mineral olivine is forced by pressure to assume a more compact form.
At \(700\ \rm km\), pressure forces some of the silicon, magnesium, iron, and aluminum into simpler oxide forms (\(\rm SiO_2\), \(\rm MgO\), \(\rm FeO\), and \(\rm AlO_2\)). The bulk of the magnesium and iron from mineral structures called magnesium silicate and iron silicate (perovskite), where silicate is \(\rm SiO_3\).
Similar chemical formulas are found in meteorites, but the minerals in the inner Earth are more compact. Most of the chemical and structural complexity of the interior is confined to the crust, where the buoyant distillates of the mantle reside and the core-mantle boundary (called the \(D^{\prime\prime}\) layer).
7.1.2. Accretion: the building up of planets#
As material is added to the forming planets by collisions, where little chucks of rock combine to make bigger rocks, the kinetic energy of impact is converted to heat. The potential energy of dust and small rocks is larger than the potential energy of material gravitationally bound to the growing planet. The lost potential energy shows up as heat, just as it does for the nebular gas going into the giant planets.
The heat added per unit of material in the outer layers of the growing planet is equal to the gravitational potential energy lost in each kilogram. The potential energy is proportional to the density and radius of the growing planet, which defines the gravitational well into which the material falls. In the early Solar System, Earth and Venus were heated the most, where the other terrestrial bodies (Mars, Mercury, and the Moon) were heated less.
Two complications are: (1) the average size of the impacting planetesimals at the end of accretion, where bigger bodies deposit their energy deeper into the growing planets; and (2) the assembly time, where longer times allow more heat from the impacts to leak out at the planetary surface and creates lower temperatures overall.
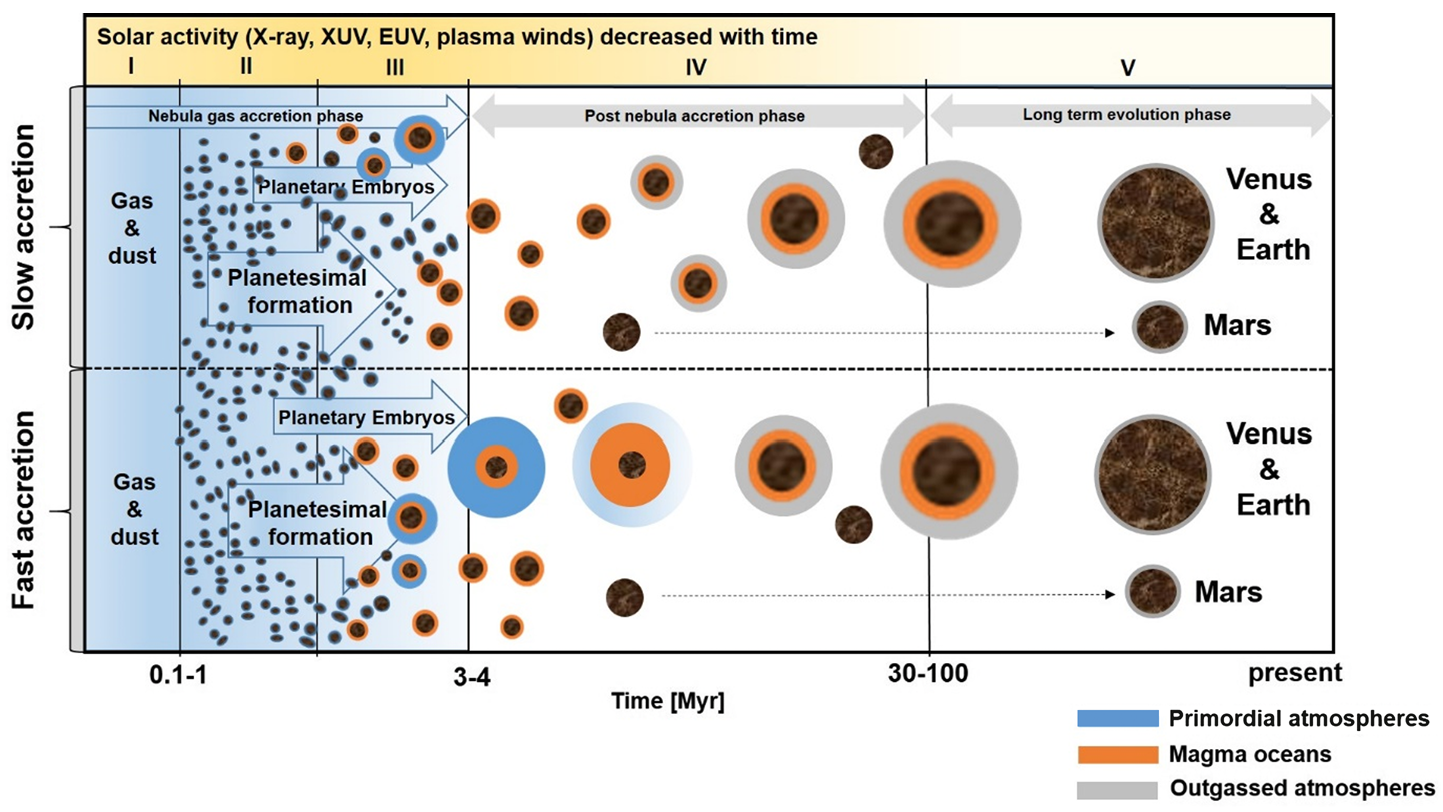
Fig. 7.3 Illustration of the five main formation stages of the terrestrial planet formation: dust settling (I), planetesimal formation (II), formation of planetary embryos (III), giant impacts including Earth’s Moon-forming event (IV). The long-term evolution phase where secondary atmospheres origin (V). Image Credit: Lammer et al. (2020).#
7.1.3. Early Differentiation after Accretion#
Earth, Venus, and perhaps Mars achieved temperatures throughout parts of their interiors above the melting point of most silicate materials by virtue of accretion. The earliest “Earth” had a massive molten region that extended from the surface down partway through the interior. Heat flowed both toward the colder center region, and outward toward space. elements that previously were bound in the solid crystalline structures of the planetesimals were free in the liquid to rearrange themselves and associate themselves with other compatible elements.
On the basis of their valence structure and the effective size of the atoms, elements can be divided into: lithophiles, siderophiles, and chalcophiles.
A lithophile (rock-loving) element tends to associate with silicate phases (note: phases are stable thermodynamic conditions).
A siderophile (iron-loving) element associates with metal phases.
A chalcophile (ore-loving) associates with sulfur-bearing phases. Chalcophile elements can be distinguished by their tendency to be volatile and escape from the solid phase.
Figure 7.4 provides the periodic table with the ionic sizes of each element. Ions significantly larger than the host magnesium or silicon ions have difficulty fitting into the solid crystal structure, and tend to stay in the molten rock.

Fig. 7.4 Ionic Radii (in Picometers) of the Most Common Ionic States of the s-, p-, and d-Block Elements. Gray circles indicate the sizes of the ions shown; colored circles indicate the sizes of the neutral atoms. Image Credit: Libretexts:Chemistry.#
In the early melting of thEarth’s outer layers, large ions (e.g., \(\rm Na\) and \(\rm K\)) tended to reside in the liquid and float to the top of this massively deep magma ocean. Earth’s crust has been geochemically cycled and processed extensively since formation, which erases the evidence for an early episode of differentiation and makes estimates for the extent of differentiation at early times controversial. We expect the early geochemical evolution on Venus to be similar (maybe even the same) as that of Earth, with less geochemical evolution occurring for smaller bodies (e.g., Mars, Mercury, and the Moon).
Only Mercury and the Moon are small enough to have undergone little crustal evolution after the initial stages of accretion, and thus may preserve the chemical evidence of that earliest part of their history. This makes sampling from the surface of the Moon or Mercury a sensible endeavor, where samples of larger bodies need to be excavated from depth (i.e., large impacts).
7.1.4. Radioactive Heating#
The building blocks of the terrestrial planets were broadly similar to the chondritic meteorites (but not identical) with more or less of the volatile elements included depending on the distance from the Sun at which particular grains condensed out. Among the elements present were uranium-235, uranium-238, thorium-232, and potassium-40, each of which are radioactive. Interestingly, both uranium and thorium have large ionic radii like potassium, and hence over time have become concentrated in the crustal rocks (particularly in granite), where the radioactive isotopes of these species average 20 ppm in abundance.
This is enough to produce \(0.03\ {\rm J}\) of energy in every kilogram of rock each year. Although this doesn’t seem like much energy, it is still substantial when the entire mass of granitic crust is considered. Roughly \(2 \times 10^{22}\ {\rm kg}\) of granite are in the crust, which leads to a total annual energy production of \(6 \times 10^{20}\ {\rm J}\), or \(2 \times 10^{13}\ {\rm W}\) of power. The present radioactivity abundances are much smaller, where estimates from mantle-derived volcanic rock suggest about 0.1 ppm and an energy production rate of \(1 \times 10^{-4}\ {\rm J/kg/yr}\). However the mantle is \({\sim}200\times\) more massive than the granitic crust, which results in \(1 \times 10^{13}\ {\rm W}\) of power from the radioactive decay of potassium, uranium, and thorium.
The effect of radioactive heating depends on a planet’s size in two ways.
The smaller the body, the less radioactive material that is present to heat the interior, and
the larger is the ratio of the surface area to volume.
As a sphere shrinks, the surface area decreases more slowly than the volume. Reduce a planet to half its original size, and the surface area drops by a factor of four while the volume drops by eight. Since more relative surface area allows faster cooling, smaller objects cool more quickly than bigger ones. Based on relative sizes Venus’ thermal history was similar to Earth’s but Mars likely cooled more quickly than Earth, and Mercury even more rapidly. We see the evidence for this in the heavily cratered surface of Mercury and in the bimodal nature of Mars, wherein both massive volcanoes and heavily cratered terrains exist.
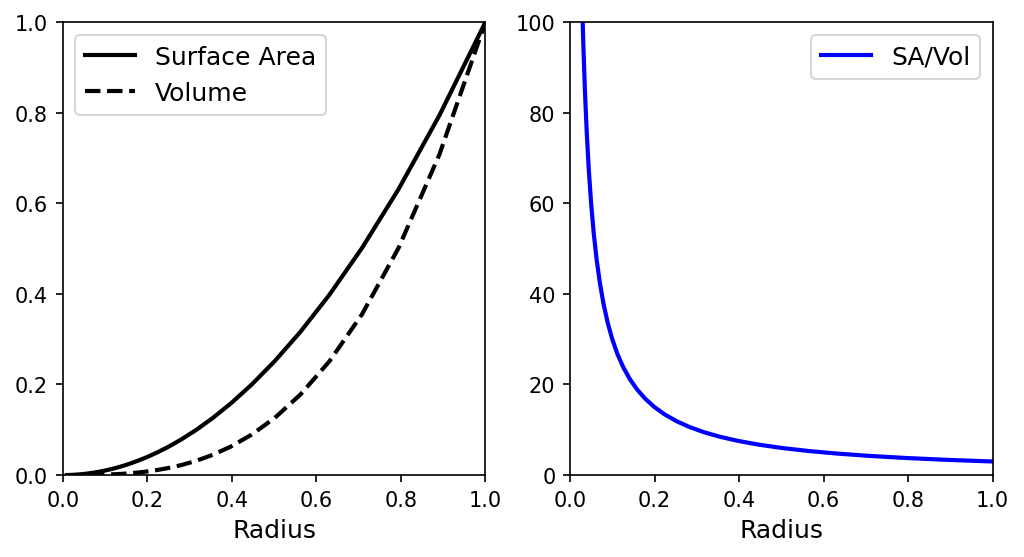
Fig. 7.5 The volume and surface area of a sphere as a function of radius (left). The surface area-to-volume ratio (right).#
import matplotlib.pyplot as plt
import numpy as np
from myst_nb import glue
fs='large'
radius = np.logspace(-2,0,41)
fig = plt.figure(figsize=(8,4),dpi=150)
ax1 = fig.add_subplot(121)
ax2 = fig.add_subplot(122)
SA = 4*np.pi*radius**2
Vol = 4./3.*np.pi*radius**3
ax1.plot(radius, radius**2,'k-',lw=2,label='Surface Area')
ax1.plot(radius, radius**3,'k--',lw=2,label='Volume')
ax2.plot(radius,SA/Vol,'b-',lw=2,label='SA/Vol')
ax1.legend(loc='best',fontsize=fs)
ax2.legend(loc='best',fontsize=fs)
ax1.set_xlabel("Radius",fontsize=fs)
ax2.set_xlabel("Radius",fontsize=fs)
ax1.set_xlim(0,1)
ax2.set_xlim(0,1)
ax1.set_ylim(0,1)
ax2.set_ylim(0,100)
glue("Volume_SA_fig", fig, display=False)
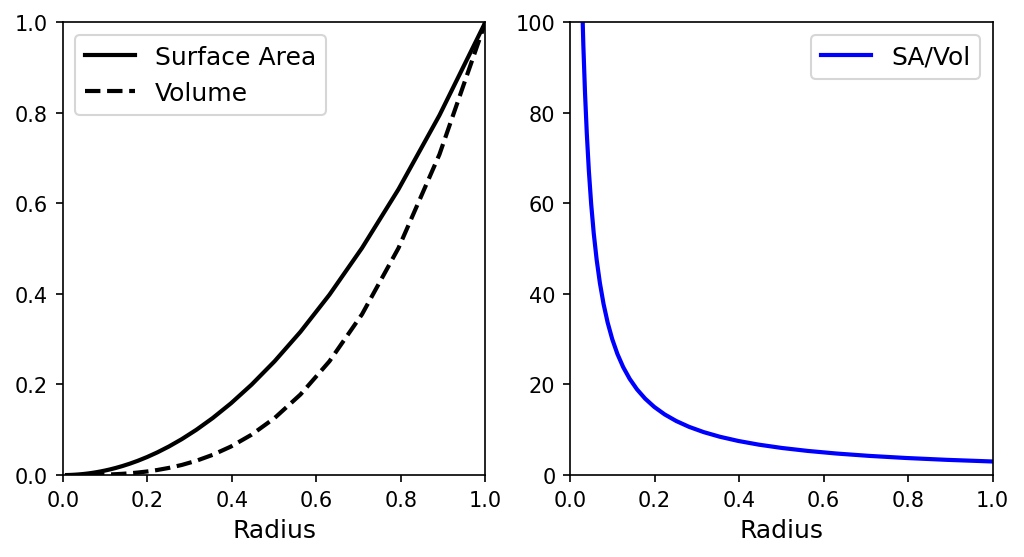
Sufficient heating is occurring today in Earth’s mantle to soften the rock and allow bulk flow to remove the heat. The Earth’s core is releasing heat to the mantle as well, so that the nature of the heat flow is somewhat complicated. Simple patterns of bulk convective motion of the mantle are interrupted by plumes of hot material driven by heat from the core. These deep-seated plumes may reach the surface in the form of large volcanoes, which are then dragged laterally by plate motion to form island chains (e.g., Hawaii).
7.1.5. Formation of the Moon#
The origin of the Moon has been a difficult issue due to its large relative size (compared with the Earth) and its low eccentricity (\({\sim}0.05\)) orbit.
Capture of the Moon after its formation is possible, but extremely unlikely because of the narrow set of conditions that could produce the orbit we see today. This includes capture into a more elliptical orbit and dissipation of eccentricity through ocean tides.
Formation in-situ with the Earth has difficulties when one tries to model the process by computer, where the small isotopic differences between the Earth and Moon are hard to explain. Moreover, the Moon lacks a substantial iron core that couldn’t be explained either.
Fission, wherein a rapidly spinning molten Earth split off the Moon, has problems with physical plausibility due to small isotopic differences. If they two bodies originated from the same parent body, we would expect almost all isotopic differences to be negligible.
Before the Apollo missions, theoretical models could not rule out completely in-situ formation or fission. The return of Moon rocks and dust samples from the Apollo missions virtually eliminated all three of the above models.
The rocks were more similar to Earth’s mantle than of primitive meteorites.
The rocks were strongly depleted in certain volatile elements relative to the Earth’s mantle, which implied more chemical processing.
In a very crude sense, one could take terrestrial mantle rocks, heat them to their vaporization temperature, and recondense only the less volatile elements to obtain lunar material. By the mid-1970s, the giant impact hypothesis emerged, which posits that the Moon is derived from the collision of the early Earth with another planet-sized body. The early Solar System would be amenable to such impacts because there was still a lot of material (planetary embryos and planetesimals) that had not yet accreted onto the final form of the terrestrial planets.
When planetesimals hit the planetary embryos or protoplanets, they would vaporize and melt resulting in the dissemination of their products into the crust of the bigger bodies. On the other hand, protoplanets at this time are not yet on stable orbits and they could collide with each other resulting in more devastating consequences. A giant impact with Uranus likely tipped that planet on its side and spun out a disk from which the moons formed. Recent studies (e.g., Ragoszinski & Hamilton (2021), Rufu & Canup (2022), Lu & Laughlin (2022)) have investigated alternative theories, but a giant impact component of the theory seems inescapable.
Detailed computer simulations show that a Mars-mass protoplanet striking the Earth could have (1) spun off a large amount of the Earth’s mantle, (2) very little iron core, and (3) a fraction of the debris would have entered a low eccentricity orbit around the Earth while the remainder was lost into orbit around the Sun or re-accreted onto the Earth.
Much of the material that shot into orbit was vaporized, with only the least volatile material remaining solid. Some recondensation occurred, but in the absence of a nebular gas providing the conditions for recondensation, much of the volatile material (e.g., water) was lost. Absence of debris from Earth’s core resulted in little iron accumulating into the Moon and the angle of impact may have facilitated the merging of cores between the impactor and the early Earth. The Moon’s present density is consistent with little or no iron.
Accretion of the material in a low eccentricity orbit to form the Moon was apparently enough to cause melting of the upper \({\sim}500\ \rm km\) of Earth’s new satellite because geochemical analysis indicates that the lunar surface is strongly enriched in lower density minerals that likely floated to the top during a molten phase. The ancient lunar highlands are especially enriched in these minerals. Higher density mineral that resemble basalts on Earth have flooded large basins on the Moon, forming the mare.
When did the Moon’s formation from Earth occur? The oldest lunar rocks found (from the highland provinces) radio-isotopically dated \(4.4-4.5\ \rm Ga\) (Borg et al. (2011)). This sets a limit on the time when the Earth’s core formed, where it had to be before the lunar-forming impact. Determining the timing and conditions of the giant impact is an active field of research using the highly siderophile elements (Jacobson et al. (2014)), orbital dynamics (Quarles & Lissauer (2015)), and numerical modeling (e.g., Canup & Asphaug (2001), Nakajima & Stevenson (2018)).
What was the origin of the impactor that struck Earth? This remains a mystery, but it is clear from the geochemistry of the Moon that the impactor had to have a composition similar to Earth. Because some of its mass went into the debris that formed the Moon, gross compositional differences would show up in the lunar rocks. Since the lunar rocks closely resemble a piece of devolatilized mantle from the Earth, the rocks could not have been very different from terrestrial mantle composition. For two bodies to collide on a timescale consistent with the dating of lunar rocks and under the right conditions (i.e., impact angle and speed), the impactor’s penultimate orbit was likely close to the proto-Earth (Quarles & Lissauer (2015)).
The enormous upheavals in the first 2% of Earth’s history are a reflection of the crowded Solar System environment at the time:
the final stages of Earth’s growth by sweep-up of smaller debris heated the planet to high temperatures, and
the apparent presence of large bodies in planet-crossing orbits that set the stage for the catastrophic collision leading to lunar formation.
7.1.6. Origin of Earth’s Atmosphere, Ocean, and Organic Reservoir#
Earth’s earliest atmosphere was a cloud of silicate vapor that surrounded it during its accretion and core formation. As accretion stopped and core formation ended, the surface cooled and the silicate vapor condensed to form molten and solid rock. If this process concluded early enough, Earth would have been surrounded by a remnant primordial atmosphere of molecular hydrogen and trace amounts of other gases. This primordial atmosphere was swept away by the strong solar wind very quickly, and is of little consequence to the rest of Earth history. Impacts were still a regular occurrence at this time so that such impacts can erode away portions of the primordial atmosphere (Kegerreis et al. (2020)) once the nebular gas had dissipated. Giant impacts are catastrophic enough to introduce many chemical species to the early atmosphere and modify the heat flow within the primordial atmosphere (Lupu et al. (2014)).
From whence came the gases that made up the “permanent” atmosphere? Outgassing from Earth’s interior could have put hydrogen sulfide, carbon dioxide, and a large amount of water (all originally dissolved in the early magma ocean) in the atmosphere and on the surface. The origin of these volatile materials may not have been in the vicinity of the forming Earth. Impactors that came from the asteroid belt (carbonaceous chondrites) and the outer solar system (comets) were rich in water ice, organics, carbon dioxide, carbon monoxide, and ammonia.
Collisions of comets with the planets would have released the cometary ices and gases into the atmospheres of the target planets. Cometary material (including water) might have been episodically added to the atmosphere. However, the ratio of deuterium to hydrogen (D/H) in the water ice portion of most comets is twice that in Earth’s ocean water. No plausible way has been found to lower the value after it has been added to the Earth. Therefore, comets do not appear to be the primary source of Earth’s water.
Bodies in the asteroid belt would have been richer in water than material near the Earth. Jupiter can perturb that material into planet-crossing orbits, which allowed for accretion by the Earth. Most of this material would have been in the form of bodies as large as the Moon, or even Mars, so that these collisions would have been violent. Nonetheless, the net effect would have been the addition of water to the growing Earth. Carbonaceous meteorites have a D/H range that averages out to the value present in the Earth’s oceans. However, some of the elemental and isotopic abundances in the carbonaceous chondrites limit to 1% the amount that could be added to the Earth. It is possible that another water-rich asteroid type existed in the past that is otherwise unknown today. Alternatively, water could have been absorbed on rocky grains closer to the Earth and brought as a gentle rain.
Comets probably brought other constituent gases (e.g., carbon dioxide, carbon monoxide, methane, ammonia, nitrogen, etc.). Carbon dioxide also could have been available from rocks in Earth’s mantle and the early atmosphere likely was dominated by this gas after the condensation of water. Molecular oxygen is essentially nonexistent in comets and is nearly absent from Mars, Venus, and the early atmosphere of the Earth.
A significant portion of the organic molecules present in the comets and meteorites may have survived intact to the surface. Thus the early ocean likely was seeded with large amounts of organic compounds, with complexity up to and including amino acids, which have been found in meteorites. As the impact rate declined and Earth’s surface began to stabilize, the materials necessary to initiate a biosphere were very likely in place.
A recent study (Catling & Zahnle (2020)) has summarized our current knowledge of the early atmosphere.
The composition of the Hadean atmosphere is obscured by a lack of well-preserved rocks, but analysis of crystals of zirconium silicate (i.e., zircons) suggests that continents, oceans, and perhaps life all originated in the Hadean.
The Hadean atmosphere and mantle were probably initially highly reducing, before subsequent oxidation either by hydrogen escape or Fe loss to the core. In any case, iron-cored impactors would reduce seawater to hydrogen and create transient, highly reducing atmospheres that may have been important for the origin of life.
At the end of the Hadean, Earth had oceans, continents, an anoxic atmosphere likely rich in \(\rm CO_2\) and \(\rm N_2\), and probably life.
7.2. Archean Climate: the Faint Young Sun#
As we have learned from Disney’s “Beauty and the Beast”, we are Certain as the Sun, rising in the East, at least as the climate goes. We base this certainty on the collective experience of a constant Sun over human history. Yet there is strong evidence that the Sun has not really shined with constant output over time, which we find from the geologic record of climate, observing other stars, and the physics of nuclear fusion. When the Sun was young, it had a lower output than today by a significant amount, leading to what is called the “faint early Sun” or “faint young Sun” problem.
There is ample evidence that the Archean Earth possessed liquid water on its surface. The existence of metamorphosed sedimentary rocks from this period require erosion by liquid water and deposition in a lake or marine environment. The presence of life itself also implies the existence of liquid water, given that most life at the time lived in aqueous environments. Today all living things require water, although some are poisoned by oxygen. With a fainter Sun, we would expect that Earth’s surface to lack liquid water and maybe be covered in ice. The geologic evidence shows that this is not the case.
7.2.1. The Faint Young Sun#
Simple reasoning about the physics of hydrogen fusion indicates that the Sun was cooler in the past than it is at present. As the Sun converts hydrogen to helium, the mean atomic weight of the atoms in the core goes up because helium is four times heavier than hydrogen, but the number of atomic nuclei goes down. As the core evolves, it compresses and forces the density up. The compression of the core towards higher density increases the average temperature.
The fusion rate is very sensitive to the temperature, where small increases in temperature can lead to a large increase in the fusion rate. This is because the average speed of the particles in the core is increased and has a larger chance of overcoming the Coulomb repulsion. If the fusion rate increases, then more photons are produced and over time the Sun has become more luminous. Computer models predict that \({\sim}3.8\) billion years ago, the Sun’s luminosity was 25% dimmer than at present (or 75% of the current value). By the end of the Archean (\(2.5\ \rm Ga\)), the Sun’s luminosity was only 18% dimmer (i.e., the Sun brightened by \({\sim}9\%\) over \(1.3\ \rm Gyr\)).
With less sunlight streaming to the Earth in the past, the surface would have been colder than at present, with everything else being equal. The Earth’s oceans today have an average temperature of \(288\ \rm K\). A simple scaling (using the Stefan-Boltzmann law) suggests that the temperature of Earth’s oceans would have been \(268\ \rm K\) at the beginning of the Archean and \(274\ \rm K\) by the end. These temperatures are near the freezing point of both freshwater (\(273.15\ \rm K\)) and seawater (\(271.15\ \rm K\)).
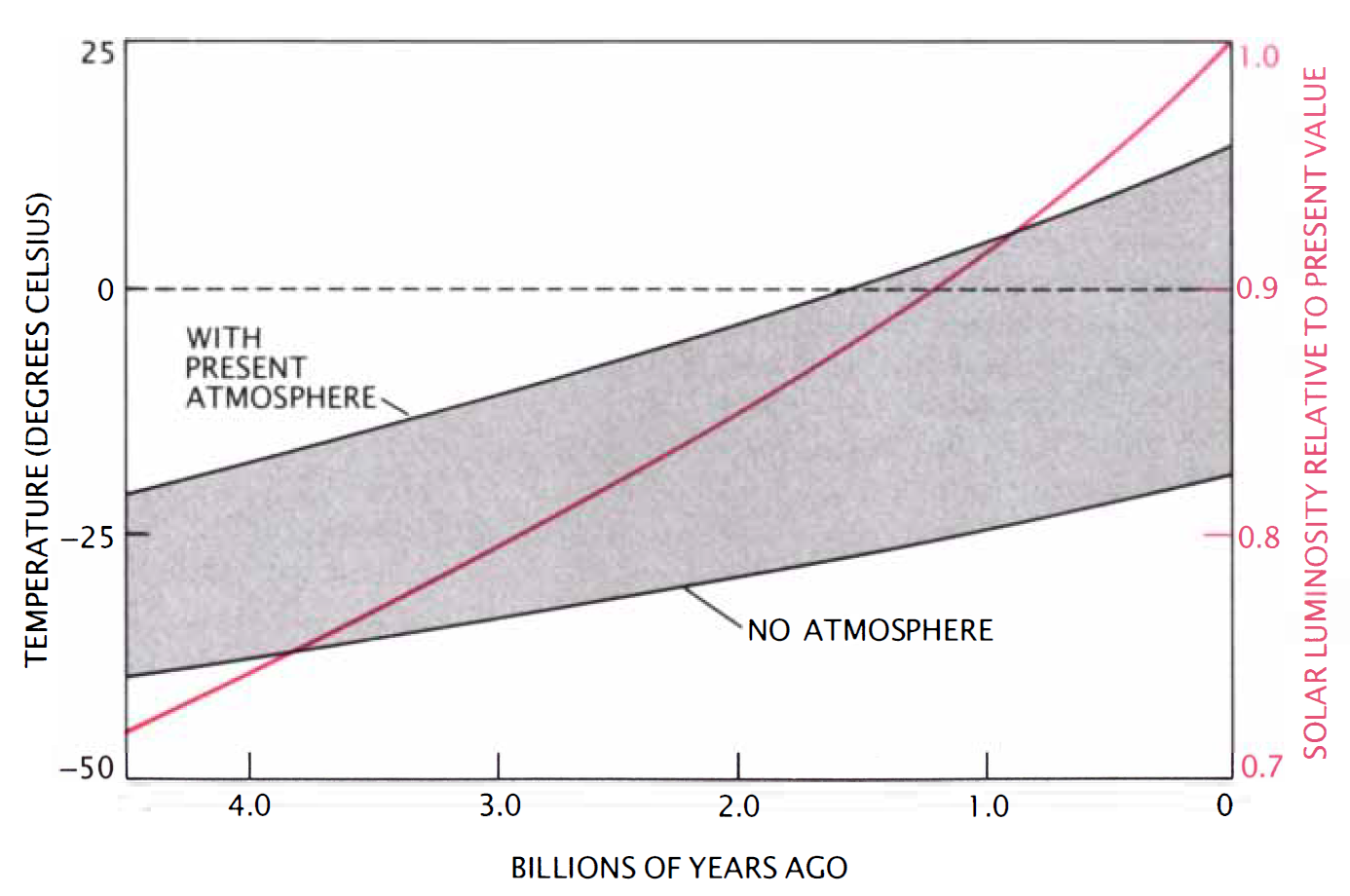
Fig. 7.6 Climate model calculations indicate that the earth would have been frozen during the first part of its history if its atmospheric composition was the same as it is today. The reason is that the sun was up to 30 percent fainter in the past (colored curve). Image Credit: Kasting, Toon, & Pollack (1988).#
As the Earth cools (from formation), the atmosphere cannot hold as much water vapor and this dryness leads to an even lower temperature through the greenhouse effect. James Kasting and colleagues have suggested that the Earth’s surface was \(255\ \rm K\) at the start of the Archean. Such an Earth would not have a stable, liquid water ocean. What kept the oceans from being frozen?
One solution is that the young Sun was not faint, but more massive and therefore as bright as today. The Sun would then need to lose enough mass over time to stabilize the climate and match the Sun’s current state. Observations do not support this hypothesis, where fast mass loss only happens in the first \({\sim}200\ \rm Myr\) after Sun-like stars form.
The energy balance for climate depends on the Earth’s reflectivity over all wavelengths (i.e., the Bond Albedo) and the greenhouse effect. Some solutions to the faint young Sun is to invoke clouds, which means:
the Archean Bond albedo was low because of substantially different cloud cover, or
abundant high-altitude cirrus clouds warmed early Earth.
However, no plausible combination of few low clouds (which increase reflectivity) and high, icy clouds (which tend to increase warming) solves the problem of the faint young Sun. Three-dimensional climate models with a lower solar flux produce weaker evaporation and fewer low clouds, but a strong greenhouse effect is still required because the albedo decrease is small (Catling & Zahnle (2020)). The most plausible solution is a big greenhouse effect, which is solved not by water vapor but noncondensable greenhouse gases, such as \(\rm CO_2\) and \(\rm CH_4\).
7.2.2. The Greenhouse Effect#
The greenhouse effect refers to the increase in temperature of the air caused by the greater difficulty that IR photons have moving outward to cooler regions, relative to the eas of movement of visible photons. (see Sect. 3.3). Its efficiency depends on the property of the medium through which the visible and IR photons move. If they are allowed through the material with equal ease, there is no resulting elevation of air temperature.
For a planetary surface like Earth’s, sunlight (at all wavelengths) streams down through the atmosphere, but some of it is reflected by clouds and the remainder reaches the surface. The visible photons are absorbed (by the ground, people, trees, buildings, etc.), where the increased vibrations of the molecules in all these things cause emission of heat (in the form of IR photons) upward through the atmosphere. But the atmosphere impedes the progress of IR photons. They are absorbed and re-emitted (in all directions) many times at many altitudes levels in their trip back up through the atmosphere. About half in each absorption/re-emission event, find themselves moving downward again. The result is an impediment to the outward flow of heat energy.
There must be a balance of incoming solar energy to outgoing thermal energy. The lower atmospheric layers have a higher temperature because they are denser and absorb photons more effectively. The absorption of IR photons causes the temperature gradient (change in temperature with altitude) to become steeper. The steeper the temperature gradient, the more efficient the flow of IR photons outward, until an energy balance is achieved. The result is an increase in the temperature at Earth’s surface by about \(33\ \rm K\) over what we would get without a greenhouse effect.
The actual situation in Earth’s atmosphere is more complicated, where clouds and surface ice very efficiently reflect roughly one-third of the solar photons back outward. About one-fourth of the solar photons are absorbed directly by clouds or the atmosphere itself. Photons do not move vertically in the atmosphere, where there is a spread in all directions due to the effectiveness of clouds to scatter the light.
The IR emission is also somewhat complicated because the ground gets several degrees warmer than the air immediately above it, which sets up convection currents (or turbulent air motions). Convection moves heat from the ground upward and continues at altitudes wll away form the ground. The rising warm air is replaced with cooler air, and the warm air loses heat through radiation of IR photons in the more transparent high layers of the atmosphere.
Turbulent motions in our atmosphere are also responsible for forming clouds. As air rises in the atmosphere, it cools and becomes less capable of retaining water vapor. Eventually, the water vapor condenses to form clouds. The process of condensation releases some energy in the form of heat (i.e., latent heat), and this must be included in the atmospheric energy balance.
Clouds are an important part of Earth’s atmospheric greenhouse process because they can cool the air (by reflecting sunlight) and heat it (by absorbing IR photons). The amount of water vapor that the air can hold is a sensitive function of temperature, so that small amounts of warming can lead to large changes in cloud cover. The rainfall produced by the clouds is the principal source of water for land-based life.
Other complications include
the daily and seasonal variations in sunlight, and
the effects of continental landmasses and ocean currents in changing the temperature and moisture content of the atmosphere.
For Earth as a whole, the net heating process that warms the surface above freezing is absorption of sunlight at or near the ground, and emission of IR photons in their upward escape, which is impeded by the absorption of the atmosphere.
7.2.3. Primary Greenhouse Gases#
Gases that cause the greenhouse effect must absorb photons in the mid-IR corresponding to wavelengths of roughly \(10-50\ \rm \mu m\). The major constituents of air (\(\rm O_2\) and \(\rm N_2\)) are poor absorbers. More important are traces species: water (\(\rm H_2O\)), carbon dioxide (\(\rm CO_2\)), and methane (\(\rm CH_4\)). Water vapor makes up no more than several percent (on average) of the molecules in the atmosphere close to the Earth’s surface, but it is highly variable over time and location. Carbon dioxide gas cannot condense to form clouds in the atmosphere and is uniformly distributed around Earth at \({\sim}0.04\%\). Methane is at a much lower abundance \(1896\ \rm ppb\) (compared to \({\sim}400\ \rm ppm\) for \(\rm CO_2\)), but its warming potential is \({\sim}30\) times higher than \(\rm CO_2\).

Fig. 7.7 This figure shows the absorption bands in the Earth’s atmosphere (middle panel) (when there are no clouds present: clouds average 60-65% cover and independently impact radiation transfer) and the effect that this has on both solar radiation and upgoing thermal radiation (top panel). Individual absorption spectrum for major greenhouse gases plus Rayleigh scattering are shown in the lower panel. Image Credit: Wikimedia Commons:Atmospheric Transmission by user Robert Rohde.#
7.2.4. Implications for Earth during the Faint Young Sun Era#
In the early Archean, the surface of the Earth would have been below the freezing point of water due to a less luminous Sun and assuming no change in atmospheric composition. But rocks dated at \(3.8\ {\rm Ga}\) are metamorphosed from sediments, which strongly suggests that running liquid water was present. The simplest way to obtain an elevated temperature for Earth’s surface is to increase the amount of greenhouse gases. Water vapor is controlled by the atmospheric temperature (i.e., the higher the temperature, the more evaporation from the ocean), and we are not free to simply invoke more water. Carbon dioxide is not so linked and given its abundance in the continental and oceanic sediments, it is plausible to explore models in which carbon dioxide was more abundant in the atmosphere.
A simple model from Kasting and Ackerman (1986) shows that increasing the atmospheric abundance of carbon dioxide increases the surface temperature. The effect is amplified because more water is evaporated from the oceans as temperatures rise, which leads to additional warming. For the Archean, the carbon dioxide abundance would necessarily be 1000 times the present value to prevent the surface water from freezing, and carbon dioxide would make up about one-fourth of the total atmosphere. To obtain the average temperature of today (\(288\ \rm K\)) requires that carbon dioxide be the dominant gas in the atmosphere, with a pressure well above 1 bar.
What is the amount of carbon available today that could have been carbon dioxide in the past? An estimate of the carbon buried in the sediments on the ocean floor and continents could yield at least a 60 bar-pressure \(\rm CO_2\) atmosphere. Hence there appears to be enough carbon locked in the crust today to maintain above-freezing temperatures in the Archean, if indeed it was in the from of carbon dioxide. However, increasing the cloud cover and removing the ozone layer can reduce the total amount of carbon dioxide needed to keep the young Earth from freezing (see Fig. 7.8)
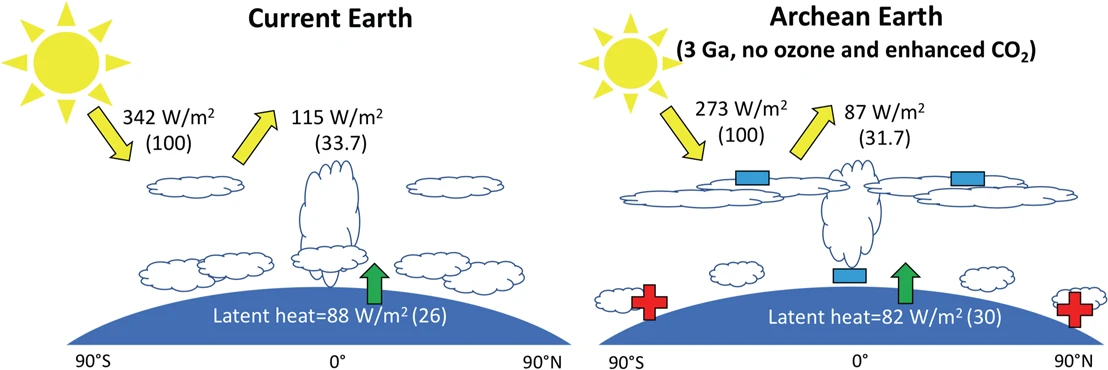
Fig. 7.8 Illustration of the change in cloud cover for the Archean Earth. The left panel shows the current Earth with global mean insolation at top of the atmosphere and reflected radiation (yellow arrows), latent heat flux (red arrow) and cloud cover. The fluxes in percent of the global mean insolation are given in brackets. The right panel shows the Archean Earth, with the insolation at \(\rm 3 Ga\), no ozone and enhanced \(\rm CO_2\) (i.e. 60 mbar) for the same global mean surface temperature as current Earth (287.9 K). Red + indicate the warming of high latitudes and blue − indicate the cooling of equatorial regions and the upper troposphere compared to the current Earth. The Archean Earth has less lower clouds and more upper clouds. Values are taken from Wolf and Toon (2013). Image Credit:Charnay et al. (2020).#
Although simplistic, the model from Kasting and Ackerman (1986) shows that the carbon dioxide level of the atmosphere was likely much higher in the past than it is today. Biogenic and abiogenic (i.e., weathering) processes have been drawing carbon dioxide out of the atmosphere over billions of years so that carbon dioxide is now a trace gas. To generalize the model, planetary scientists have added a range of feedbacks, sources, and sinks so that the carbon dioxide level better matches the geological record.
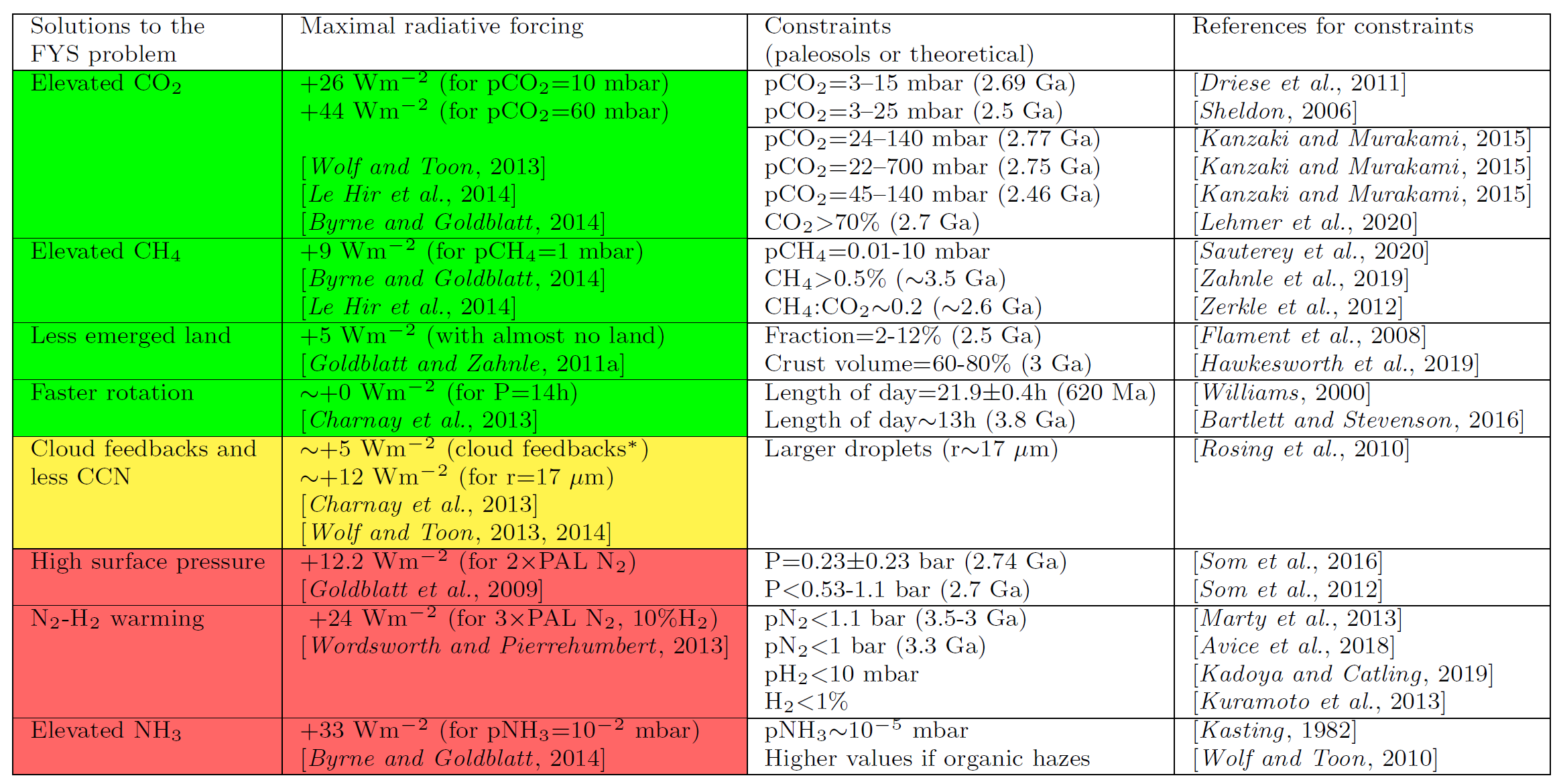
Fig. 7.9 Table of solutions to the Faint Young Sun Problem. Image Credit:Charnay et al. (2020).#
7.2.5. Paleosols and the Carbon Dioxide Abundance#
As rock are attacked by water during erosion and weathering processes, elements embedded with the crystal structures of the minerals become mobile and may move to other locations. They may form new chemical compounds, and the nature of these compounds can be a sensitive function of the ambient conditions. Paleosols are soils that are preserved through burial and hardening (without extensive metamorphism), may preserve an indirect record of the composition of the atmosphere at the time the weathering occurred.
At present, molecular oxygen is the second most abundant gas in our atmosphere. Its presence and reactivity have a profound effect on rock weathering (through oxidation and rust formation). Rocks occurring prior to about \(2\ \rm Ga\) show evidence of ferrous oxide (\(\rm FeO\)) being mobilized by weather processes. This relatively oxygen-poor compound of iron requires that the atmospheric oxygen abundance at the time be very low. Ferrous oxide is readily dissolved in water, mobilized, and becomes available to combine with other minerals that are sensitive to atmospheric conditions.
Some iron-rich basalts that were weathered to soils in oxygen-poor conditions (i.e., the Archean to early Proterozoic) have lost much of their iron through reaction with water. Some of the iron probably ended up in the local ground-water table, but some reacted with silicates to form iron silicates such as greenalite. The formation of such silicates is not possible with large amounts of carbon dioxide in the atmosphere. The carbon dioxide, diffusing into the soils, would force the formation of an iron carbonate, siderite. Thus, the absence of iron carbonate in paleosols formed in oxygen-poor conditions sets a limit on the abundance of carbon dioxide.
Analysis of paleosols from Canada shows no evidence of iron carbonates, where iron silicates are present instead. The formation of iron carbonates is sensitive to temperature, and the carbon dioxide concentration in the soils was not exactly the same as that in the atmosphere. Despite these uncertainties, it is possible to estimate a carbon dioxide upper limit. A maximum of 3% atmospheric \(\rm CO_2\) existed at the time of the paleosol formation , or 100 times the present value. This upper limit depends on the assumed soil temperature, where lower temperatures would result in a lower upper limit. The upper limit can fall to \(1-10\) times the present atmospheric value of \(\rm CO_2\) by about one billion years ago.
Could it be that enhanced carbon dioxide was not the sole contributor to a thicker greenhouse atmosphere? Other gases, particularly methane, are believed to present and contributed additional IR absorption. Some models fo the early Earth atmospheres predict methane concentrations between \(100-1000\) times the current value. Methane is easily broken apart by sunlight in the presence of oxygen in modern Earth’s atmosphere, where the lack of molecular oxygen tends to stabilize methane.
Another possible greenhouse gas is ammonia (\(\rm NH_3\)), which is extremely unstable to breakdown by sunlight. However, a shield of aerosolized hydrocarbons (similar to Titan’s atmosphere) might have blocked UV radiation from reaching most of the ammonia, thus extending its lifetime. Through a 3D Global Circulation Model, Charnay et al. (2013) show that only \(100\ \rm mbar\) of \(\rm CO_2\) and \(2\ \rm mbar\) of \(\rm CH_4\) are necessary for a temperate climate to form on the Archean Earth. Thus, a more complex atmospheric composition is probably in required to solve the faint young Sun paradox. Finally, the role of clouds in the energy balance of the Earth’s atmosphere also remains to be properly quantified, but this is extremely difficult to do given that their role in the present climate is very difficult to treat (recall that clouds can warm or cool).
7.2.6. Carbon Dioxide Cycling and Early Crustal Tectonics#
How did a thicker carbon dioxide atmosphere dwindle away over time? Carbon dioxide is taken out of the atmosphere by weathering of rock and by photosynthetic organisms (e.g., plants and bacteria). Much of the biologically trapped carbon continues to cycle through living organisms at the surface. However, some of the carbon dioxide ends up in shell-forming organisms, which die and drop to the ocean floor becoming part of the sediment and effectively removing the carbon from circulation in the biosphere. The chemistry of the weathering process is discussed in Section 4.3.1.
The breakdown of silicate rocks by the weathering action of rainwater is very efficient, because carbon dioxide gas dissolves in the rainwater to make a weak acid (similar to soda water) that can attack the rock chemically. This yields silicate, hydrogen carbonate ions, and calcium ions. The ions are quite reactive and combine to form calcium carbonate shells. The shells are preserved as thick layers of sediment on the floors of lakes, seas, and the ocean. The coral reefs are a more spectacular example of structures formed from the deposition of calcium carbonate.
How long would it take this process to remove all carbon dioxide from the present atmosphere? At current weathering rates and total biota in the oceans, the removal time is less than one million years. By comparison, this is only \(0.02\%\) of the whole history of Earth. With most of the carbon dioxide gone, the oceans would freeze over very quickly. Something else must happen (today and in the past) to release the carbon dioxide from the calcium carbonate and return it to the atmosphere.
Plate tectonics is that something, where the ocean floor is continually recycled back into the mantle at subduction zones, and the carbonate-laden sediments are carried with it. Carbon-bearing materials react with silicates at high temperatures to produce calcium silicates and \(\rm CO_2\) gas. The carbon dioxide gas emerges at the subduction zones where volcanism is occurring. Volcanoes are continuously belching \(\rm CO_2\) gas from deep within the subducted plates and resupplying the atmosphere.
At current plate-tectonics spreading rates, the carbon cycle takes about \(60\ \rm Myr\). Any given carbon atom in atmospheric carbon dioxide typically will form carbonate, be subducted, and then released again as carbon dioxide gas in a time of order \(60\ \rm Myr\). Table 7.1 lists some of Earth’s carbon reservoirs with measured or estimated abundances in billions of tons (or gigatons of carbon, \(\rm GtC\)). The atmospheric reservoir is mostly carbon dioxide, and the oceanic reservoir is mostly hydrogen carbonates, both of which are dwarfed by the carbonate sediments on the ocean floor.
Reservoir |
Amount (in \(\rm GtC\)) |
---|---|
Atmosphere |
720 |
Ocean |
38,000 |
Carbonates in sediments |
\(\geq 6 \times 10^7\) |
Biomass (alive) in biosphere |
600 |
Biomass (dead) |
1200 |
Fossil fuels |
4100 |
\(1.5 \times 10^7\) |
|
Inorganic carbon |
38,000 |
7.2.6.1. Negative Feedbacks in the Carbon-Silicate Cycle#
The carbon-silicate weathering cycle might well have a stabilizing influence on Earth’s climate because the cycle requires liquid water to dissolve carbon dioxide gas and to effectively weather rock. A fully frozen Earth would have lost the erosive portion of the process. Carbon dioxide gas would not be lost to the ocean floor, while more carbon would continue to accumulate in the atmosphere. This would raise the temperature through the greenhouse effect, until the oceans could melt and liquid precipitation could resume.
Higher atmospheric temperatures increases the evaporation from oceans, the amount of cloud present, and hence rainfall rates. Higher temperatures also favor rainfall over snow at high latitudes and elevations. These have the net effect of increasing the rate of weathering by rainfall, and hence removal of carbon dioxide from the atmosphere. Sedimentation rates are higher, but the rates of subduction and carbon dioxide outgassing are not affected. As a result, carbon dioxide abundance in the atmosphere decreases, weakening the greenhouse effect and lowering the temperature.
The carbon-silicate cycle possesses a negative feedback loop in which changing the conditions in one direction tends to cause the system to move back in the other direction. This is characteristic of physical systems that are stable and provides at least a partial explanation as to why Earth’s climate has remained in the temperature range allowing for liquid oceans over geologic time. Without such a feedback, changes in the Sun’s luminosity, the plate-spreading rate, and the amount of volcanism could have moved Earth’s climate out of the range in which life is sustainable.
Life itself has played a role in altering carbon loss rates:
the development of soil-forming microorganisms some \(3\ {\rm Ga}\) accelerated the trapping of \(\rm CO_2\) into soils and my have led to a net decrease in carbon dioxide levels in the late Archean atmosphere.
the development of plankton containing calcium carbonate shifted most of the deposition of carbonate to deep ocean rather than to shallow, continent-shelf environments, hastening the transport of carbonates to subduction zones and increasing the rate of carbon dioxide returning to the atmosphere.
7.2.6.2. The Carbon-Silicate Cycle during the Archean#
Although the carbon-silicate cycle seems to be a good candidate for explaining how Earth’s climate has been stabilized over time, it is necessary to think carefully about how it operated during the Archean.
Smaller amounts of continental mass was exposed above sea level, which could have reduced the efficacy of silicate weathering. This step determines the rate of carbon dioxide sequestration.
More rapid recycling of crust at the time would have led to a faster return of carbon dioxide to the atmosphere. A consequence would be less carbon dioxide stored in sediments on the seafloor.
Theres two speculative possibilities between the carbon-silicate cycle in the Archean and modern times mitigate in favor of leaving the available carbon dioxide in the atmosphere rather than locking it into sediments.
The high carbon dioxide abundances required to sustain Archean temperatures above that of the water freezing point led to a potential instability in the early Archean. The Sun’s luminosity was low enough that feedbacks in the carbon-silicate cycle might not have worked to bring earth out of an ice-covered (snowball) state. Cold temperatures and high carbon dioxide abundances would have caused carbon dioxide clouds to form, with the cooling effect of these clouds short-circuiting the ability of the gas to warm the surface. This is a problem unique to the Archean because for higher solar luminosities, less carbon dioxide is required to drive Earth out of a global ice age.
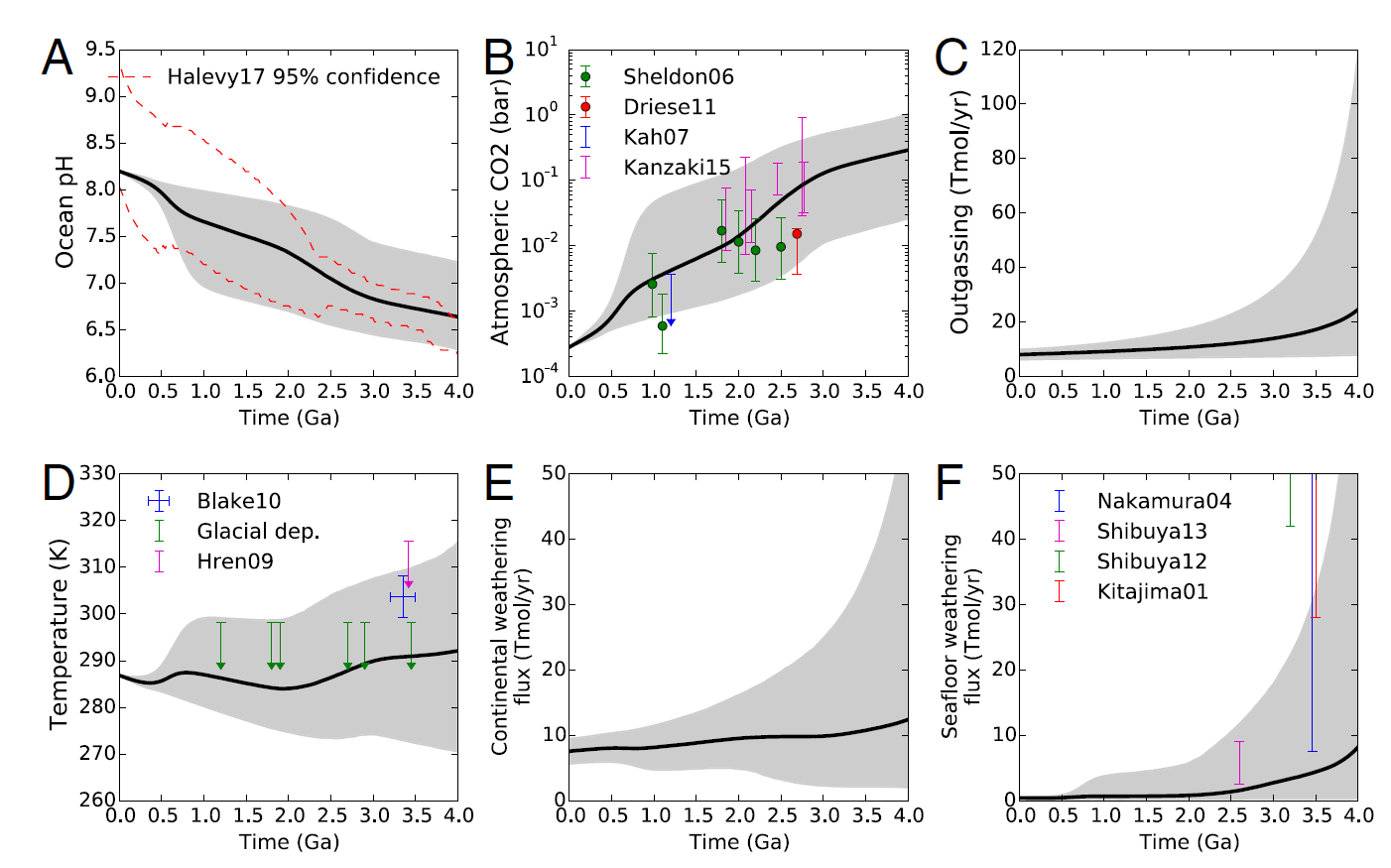
Fig. 7.10 Models showing the changes in (a) ocean \(\rm pH\), (b) atmospheric \(\rm CO_2\), (c) outgassing, (d) mean surface temperature, (e) continental weathering, and (f) seafloor weathering across geologic time. The gray shaded regions represent the 95% confidence intervals, and black lines are the mean outputs. Image Credit:Krissansen-Totton, Arney, & Catling. (2018).#
7.3. Homework#
Problem 1
Explain how we can determine the internal structure of the Earth. Can similar methods be applied to our exploration of other bodies within our Solar System?
Problem 2
Describe how accretion and early differentiation influence the final makeup of a planet. How would the Earth be different if all accretion ended while Earth was in its molten phase?
Problem 3
Radioactive heating is a huge source of energy for a young planet. How does the is energy source change over time? How does the cooling rate differ based on planetary properties?
Problem 4
Summarize our best explanation for the formation of the Moon. How did the sample return from the Apollo missions rule-out the other potential hypotheses?
Problem 5
Describe how the early Sun (\({\sim}4\ \rm Ga\)) was different than our current Sun. How would these differences have affected the prospects of life surviving on the surface.
Problem 6
Summarize how the greenhouse effect is used to address the faint-young Sun problem.
Problem 7
Explain how we can use paleosols (ancient soils) to understand the ancient climate of the early Earth.
Problem 8
The development of life on the early Earth transformed the atmosphere and produced a feedback within the Carbon-Silicate cycle. Describe this feedback and how it affected the global climate.