Early Earth
Contents
6. Early Earth#
6.1. Evolution of Metabolism and Early Microbial Communities#
The process of metabolism is a hallmark of all living organisms. Catabolic reactions generate energy for the organism while anabolic reactions are used for the synthesis of cell material. Metabolic pathways in today’s living organisms have been evolving for more than \(3.5\ {\rm Gyr}\). The evolution of metabolism cannot be separated from the origin life because it was necessary for even the earliest organisms. Contemporary metabolic pathways are presumed to be much more elaborate and sophisticated that those first evolved. Metabolism today ranges from
the use of various inorganic chemicals (e.g., hydrogen or sulfur for energy),
several forms of photosynthesis, and
metabolism of hundreds of organic compounds.
It is presently impossible for us to know which pathways originated first and how they evolved. Understanding how metabolism evolved on the early Earth is of considerable importance. Microorganisms resembling today’s Bacteria and Archaea were most likely the first organisms, and the evolution of their metabolism is the most relevant. Identifying the early evolution of metabolism in these organisms enables us to distinguish specific metabolic types from the later innovations.
6.1.1. The Setting: Conditions on Early Earth#
On the early Earth, there were physical and chemical conditions that influenced the evolution of metabolic processes.
One factor that influenced physical conditions was an instability in the environment (e.g., large changes in available sunlight, water, or temperature). An impact of an asteroid (\(R{\sim}50\ {\rm km}\); see Fig. 5.16) can sterilize all surface life on our planet and evaporate a global ocean that is \(3\ {\rm km}\) deep. Only microbial ife at a depth of several \({\rm km}\) could then survive. Cosmic events affected early life in major and largely unknown ways that may have lead to the extinction of many species and redirection of the evolution of Earth’s biota. It is possible that catastrophic events during the early history of Earth may have also affected the evolution of metabolism.
Chemical conditions on early Earth were marked by a relative dearth (absence) of nutrients that make life so rich today. A steady supply of certain nutrients was available, which may have influenced the emergence of specific metabolic pathways. Organic and other volatile materials are synthesized in space and became incorporated into the Earth through impacts by large bodies. These impacts may have disrupted evolution, but also provided nutrients for life. In addition, the abiotic synthesis of organic molecules occurred on Earth. Acetic acid (\(\rm CH_3COOH\)) synthesis has been shown to occur under primordial conditions.
Although on the early Earth organic materials were less abundant than today, we may reasonably assume that they were present in sufficient quantities and varieties for life to originate and evolve. Organic materials alone would not likely have been sufficient to sustain life. Other nutrients were necessary for organisms to utilize electron donors and acceptors to generate metabolic energy needed for life (see Table 6.1). The first metabolisms that arose were carried out anaerobically due to the lack of free oxygen.
Nutrient |
Sources on primitive Earth |
---|---|
Fixed Carbon |
|
Simple amino acids, sugars, other organics |
Meteors and asteroids |
Acetic acid |
Chemosynthesis on Earth |
Electron donors |
|
Hydrogen (\(\rm H_2\)) |
Volcanic outgassing |
Ferrous Iron (\(\rm Fe^{2+}\)) |
Mineral dissolution |
Electron acceptors |
|
Carbon dioxide and monoxide (\(\rm CO_2\) and \(\rm CO\)) |
Volcanism |
Sulfite (\(\rm SO_3^{-}\)) |
Volcanism; hydration of sulfur dioxide (\(\rm SO_2\)) |
Elemental sulfur (\(\rm S\)) |
Mineral dissolution |
6.1.2. Evidence for the Nature of Early Metabolisms#
A few categories of more direct evidence can elucidate the nature and timing of early metabolisms. This evidence comes from three primary sources: biomarkers, the geochemical record, and phylogenetic evidence.
Unique biomarkers have been useful for understanding early metabolisms. For example, hopanoids are found only in cell membranes of the bacterial group cyanobacteria, which carryout oxygen-producing photosynthesis. The presence of hopanoids has been used to date the evolution of these organisms to about \(2.5\ {\rm Ga}\). This date agrees well with the geochemical record for oxygen production on Earth and supports the view that the first oxygenic photosynthetic organisms were cyanobacteria.
The geochemical record has been useful in establishing evidence for certain types of metabolic activity. Strong evidence indicates that carbon dioxide fixation was an early metabolic event on Earth, which is called primary production. This is when environmental \({\rm CO_2}\) is taken up and the carbon is incorporated into organic matter. Carbon dioxide fixing organisms cause isotopic fractionation. As a result in the Isua deposits in Greenland (that date to \(3.5\ {\rm Ga}\)), deposited organic carbon has a lighter isotopic signature than carbonate.
Although this is excellent evidence that primary production occurred on early Earth, there is no definitive evidence about the nature of the earliest primary producing organisms. Were they using chemical energy (chemosynthetic) or light energy (photosynthetic), and which organisms were responsible for this process? The anaerobic methane oxidizing microbes are known to produce the lightest organic carbon, which may explain the existence of lighter organic carbon isotopes in certain layers of the Isua sediments.
Phylogenetic analysis is another useful approach for identifying which bacterial groups were involved in early metabolism. By knowing which group of organisms evolved first, it is possible to hypothesize which metabolic activities arose first. The deepest branches of the RNA Tree of Life are of thermophilic Archaea and Bacteria, implying that these are the earliest ancestors of any organisms still alive today. However, two issues are important:
discernible detail regarding the major groups of microorganisms is in adequate, so there remains a question about which organisms might have been the earliest; and
horizontal gene transfer, which involves the exchange of genes among different lineages.
Most genes are transferred by vertical inheritance from one generation to another within a species.
Some of the genes responsible for carrying out methanogenesis (methane production) have also been found in bacteria that are methane consumers. Methanogens are found in the domain Archaea, whereas methane consumers are found in the domain Bacteria. This sort of horizontal gene transfer makes it very difficult to trace the evolution of metabolic functions and other characteristics of organisms.
6.1.3. Contemporary Metabolisms#
The evolution of life has changed the Earth, even as the Earth has provided the habitats for life. For example the volution of oxygenic photosynthesis gave rise to oxygen on Earth and enabled the later evolution of aerobic metabolism. To understand the evolution of metabolism, we study the essential metabolic features of present-day organisms.
6.1.3.1. Anabolism#
One of the two major parts of metabolism is anabolism (i.e., constructive metabolism or biosynthesis), which is responsible for the building of new cell material for growth and reproduction. The heterotrophs synthesize cell material from organic molecules that are present in their environment. In contrast, the autotrophs make organic molecules from carbon dioxide in a process called carbon dioxide fixation. The autotrophs are important because they are the primary producers that ultimately provide the entire biosphere with organic material.
The most advanced pathway for carbon dioxide fixation is the Calvin-Benson cycle used by plants and some bacteria as part of photosynthesis. Other pathways include the acetyl coenzyme A, reverse tricarboxylic acid, and hydroxypropionate pathways.
6.1.3.2. Catabolism#
The chemical reactions that constitute metabolism must be thermodynamically feasible. Anabolic reactions require energy to create order, and thus organism must also have catabolic pathways (i.e., destructive metabolism) to generate energy needed for anabolism. Catabolic pathways are remarkably diverse, where some organism “burn” organic food using oxygen (i.e., aerobic organotropy). Before oxygen, organisms “breathed” substances such as carbon dioxide, sulfate, iron, and other substances. The lithotrophs “eat” hydrogen, sulfur, ammonia, and other unlikely-seeming foods. The phototrophs harvest the energy of light.
6.1.3.3. Oxidation, reduction, and electron flow#
Catabolism follows the principles of oxidation and reduction. These principles are best illustrating using a form of catabolism called respiration. For respirers, oxidation corresponds to “eating” as reduction is to “breathing.” Between the two types of reactions, electrons flow and the work done using catabolic energy is analogous to the work done by electrons flowing between the terminals of a battery. The only requirement is that the reaction has the potential to release energy, which condition is realized if the substance oxidized has a more negative electrical potential than the substance reduced.
To generate energy the organism oxidizes (or “eats”) the electron donor, while it reduces (or “breathes”) the electron receptor.
Respirers range from methanogens and acetogens, which utilize carbon dioxide and produce methane and acetic acid as waste products, to aerobes (which use oxygen and produce water). The electron acceptor has also been a major constraint in the evolution of catabolism because electron acceptors (e.g., oxygen) became abundant relatively late.
6.1.3.4. Respiration, photosynthesis, and fermentation#
The three forms of catabolism are respiration, photosynthesis, and fermentation, which are distinguished largely by their patterns of electron flow. Respiration is the most straightforward because separate substances in the environment (i.e., substrate) are used by organism as electron donor and acceptor. In photosynthesis and fermentation, electrons still flow from a donor to an acceptor, but the pattern is less intuitive.
Photosynthesizers take advantage of the key property of chlorophyll, which functions well as an electron acceptor and an effective electron donor (if energized by light). As a result, electrons flow from and to chlorophyll in a cyclic pattern, doing work in the process. Superimposed on this chlorophyll-driven cyclic electron flow, electrons from an electron-donating substrate are used to reduce carbon dioxide in carbon dioxide fixation. Fermenters have a pattern, where an electron-donating substrate is oxidized and the electron acceptor is an internal metabolic intermediate rather than a substrate.
6.1.3.5. ATP and the proton-motive force#
Catabolism consists of metabolic pathways that involve oxidation and reduction. How is the energy actually stored and transferred? Adenosine triphosphate (ATP) is the energy currency of biology, ATP contains phosphoanhydride bonds that yield energy upon hydrolysis (i.e., breakdown through combination of water). Certain other compounds have energy-rich bonds too, including adenosine diphosphate (ADP), pyrophospate (PP), and other metabolic intermediates. ATP predominates in present-day organisms.
The formation of ATP and the hydrolysis of ATP provide the energy link between catabolism and anabolism, where the former generates ATP and the latter uses ATP. The mechanism of ATP generation is usually simplest in the case of fermentation, where ATP is directly generated by reactions that are part of a metabolic intermediate containing an energy-rich phosphoanhydride bond that transfers its phosphate to ADT and forming ATP.
For respiration and photosynthesis, ATP is generated in a two-stage process in which the cell membrane plays an essential role. Just as a membrane is necessary to separate the contents of a cell, so is a membrane necessary for energy generation and storage. The principle is akin to charge separation.
The flow of electrons through a membrane-bound electron transport chain crates a potential between the interior and exterior of the cell.
Protons move across the potential due to the electromotive force and gradient in pH (proton concentration). This movement of protons is called the proton-motive force within biology.
The energy supplied by the proton-motive force is then used to make ATP, via a membrane-bound enzyme called ATP synthase that couples the synthesis of ATP to the import of protons.
This process is termed electron transport phosphorylation or oxidative phosphorylation. Hence the cell membrane plays a central role not only in the cell’s structural integrity, but also in its ability to generate energy.
6.1.3.6. Energy yields of catabolism#
All catabolic mechanisms lead to the generation of ATP. But which forms of catabolism generate the most ATP and are the most advantageous for the organism? A quantity used by chemists to evaluate the energy yield of a reaction is the change in Gibbs free energy (\(\Delta G\)) expressed in kilojoules (\(\rm kJ\)).
A large negative \(\Delta G\) indicates a high yield of energy, while a positive \(\Delta G\) signifies that energy is consumed. Hence, all catabolic pathways must have a negative \(\Delta G\), where the more negative the better. The \(\Delta G\) depends on the relative electrical potential of the electron donors and acceptors.
Aerobic respiration (ATP yield \(=38\)) using glucose (\({\rm C_6H_{12}O_6}\)) as an electron donor and oxygen as an electron acceptor is one of the most effective in producing ATP:
Aerobic respiration produces a large negative \(\Delta G\) because the oxidation of glucose occurs at a very negative redox potential, while the reduction of oxygen occurs at a very positive redox potential. Organisms that carry out aerobic respiration can grow fast, which includes highly evolved forms such as animals.
Photosynthesis is effective through the light-driven electron flow via chlorophyll and appreciated when one realizes that oxygenic photosynthesis is the reverse of aerobic respiration. Since the reverse of an energy-yielding process is an energy-requiring one, the use of light via chlorophyll must be very effective. Like animals, plants are also highly evolved and successful.
Other forms of respiration (e.g., methanogenesis or sulfate reduction) yield less energy:
Although methanogens and sulfate reducers use electron donors with a large negative redox potential (e.g., hydrogen or organic substances), the electron acceptors (carbon dioxide and sulfate) have redox potentials that are also in the negative range. Thus, the \(\Delta G\)’s have small negative values and the ATP yields are low.
Fermenters are also limited by small redox potential differences between the electron donor and acceptor. Lactic acid fermentation (ATP yield \(=2\)) has a low ATP yield and \(\Delta G = -196\ {\rm kJ}\). Fermenters also lack the opportunity to fully oxidize their substrates.
6.1.4. Early Metabolic Mechanisms#
Metabolism today is complex and diverse. One of our tenets is that life, and metabolism, began simpler and more uniform. The continuity of evolution means that all contemporary metabolism evolved from these modes beginnings. Our other tenet is the early life had to make do with the nutrients that were available.
Oparin originally hypothesized that the first metabolisms were not photosynthetic, where organisms obtained organic carbon from their surroundings rather than synthesizing it photosynthetically from carbon dioxide (i.e., heterotrophic). Photosynthetic organisms are much more complex than heterotrophic organisms because they need pathways to carryout out photosynthesis and corban dioxide fixation. This is in addition to the anabolic pathways for synthesis of amino acids and other cell materials. In contrast, heterotrophic metabolism requires relatively few enzymes to produce cell material from organic precursors.
A simple type of catabolism is the Stickland reaction that occurs in certain bacteria and involves the fermentation of amino acid pairs. In this reaction, only two amino acids are needed and very few enzymes are required for catabolism and generation of ATP. It is interesting to note that alanine and glycine in examples of the Stickland reaction are very stable and commonly found in carbonaceous chondrite meteorites. Therefore, these substrates may have been available for metabolism early on.
The hypothesis that heterotrophic metabolism arose first ignores the importance of primary production (i.e., the production of organic material either by chemosynthesis or photosynthesis). Heterotrophic organisms require preformed organic material. Although organic substrates may have been abundant on early Earth, within a short time they would have been depleted by any heterotrophic organisms. Some process in which organic material is produced biologically on Earth is of fundamental importance in early metabolism. The most important question concerning early metabolism becomes: What were the most likely metabolism(s) of the initial primary producers?
6.1.5. Evolution of Methanogenesis and Acetogensis#
6.1.5.1. Today and on the early Earth#
Methanogens and acetogens partly share analogous metabolic pathways, although not phylogenetically related. Methanogens and acetogens are both anaerobes that specialize in metabolism using one-carbon intermediates. Hydrogen is a common electron donor and carbon dioxide a common electron acceptor. Methane and acetic acid are the respective catabolic products. Both organisms fix carbon dioxide by the acetyl coenzyme A (\(\mathbf{\rm CoA}\)) pathway. Today methanogens and acetogens play essential roles in anaerobic ecosystems and live in a variety of habitats ranging from hydrothermal vents, pond sediments, and animal digestive tracts.
Note
A coenzyme is any non-protein molecule required for the functioning of an enzyme. Coenzyme A is a specific sulfur-containing organic coenzyme.
Today, methanogens in sediments team up with a variety of fermenters to convert cellulose-type materials to methane and carbon dioxide. Before photosynthesis, the Earth and its biosphere were quite different. Organic materials were limited to those formed by abiotic processes and by a feeble primary production. Autotrophy (or primary producers) may have been preferred over heterotrophy, necessitating a means of carbon dioxide fixation. On the early Earth, electron donors and acceptors for catabolism may have been rare or limited to a few elements or compounds. In this most ancient of biological worlds, methanogens or acetogen may have been the only metabolic types.
Analogs of the early Earth may exist today:
Microbial communities in the Earth’s subsurface are apparently dominated by methanogens that obtain hydrogen from reduced minerals in rock.
Deep basalt aquifers, hot springs, and submarine hydrothermal vents are examples of sites where microbial ecosystems may be supported by hydrogen of geological origin.
These environments that are poor in organic material but potentially rich in mineral nutrients could resemble ecosystems that dominate on the early Earth or that may exist on other planetary bodies.
6.1.5.2. Metabolic simplicity and the acetyl CoA pathway#
It is reasonable to assume that the earliest organisms were also the simplest. Frequent bombardment of the early Earth might have limited the time available for complexity ot evolve. From this standpoint, methanogenesis and acetogenesis are appealing as the earliest metabolisms. In its simplest form, the metabolisms of methanogens and acetogens each consist of three essential pathways.
reduction of carbon dioxide to a methyl (\(\rm CH_3\)) group,
the acetyl coenzyme A (\(\rm CoA\)) pathway that uses the methyl group to form \(\rm CH_3CO-CoA\), and
a single energy-yielding step.
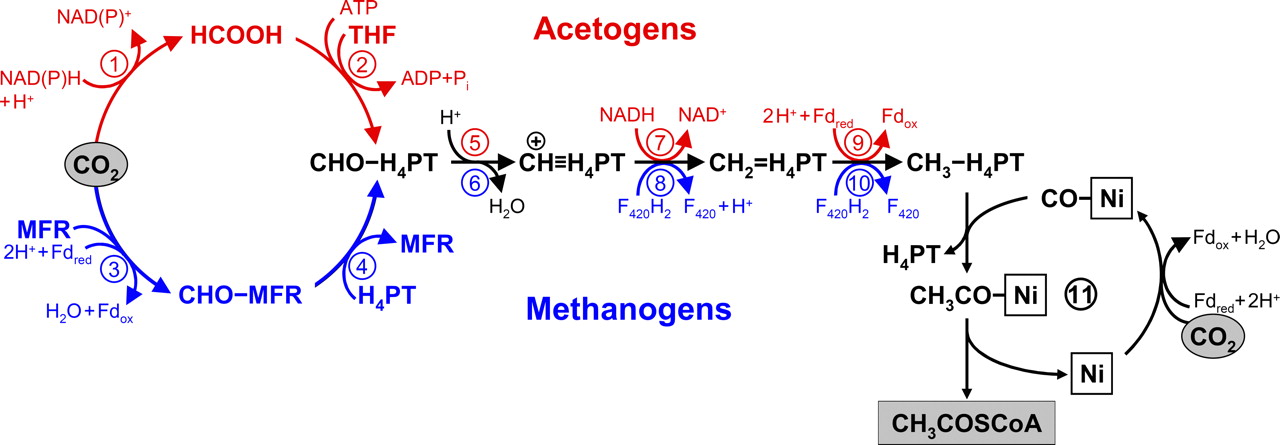
Fig. 6.1 The reductive acetyl-CoA (Wood-Ljungdahl) pathway. The upper part (red) shows the variant of the pathway functioning in acetogens, and the lower part (blue) depicts the pathway in methanogens. Image Credit: Berg (2011).#
Methanogens reduce additional methyl groups to methane, while acetogens convert some of their acetyl \(\rm CoA\) to acetic acid and gain an ATP in the process. The acetyl \(\rm CoA\) pathway may have been the crux of an ancient autotrophic mode of life. Other pathways of carbon dioxide fixation are more complex, involving various numbers of multi-carbon intermediates. Because of its simplicity the acetyl \(\rm CoA\) pathway may have been the easiest to evolve.
6.1.5.3. A prebiotic precursor for acetyl COA pathway#
Further support for the ancient nature of the acetyl \(\rm CoA\) pathway comes from evidence that the crucial reaction could have occurred prebiotically. Günther Wächtershäuser proposed the theory that metabolism evolved from prebiotic chemistry, where he has shown in the laboratory that under primordial conditions a methyl group can react with carbon monoxide to yield an acetyl group (Huber and Wächtershäuser (1997)).
Particularly interesting was the observation that the reaction required nickel sulfide and iron sulfide as catalysts. The enzyme that catalyzes the reaction in present-day organisms also contains a nickel-iron-sulfur center. A core step in the metabolism of methanogens and acetogens may have derived from a prebiotic chemical reaction. Wächtershäuser has suggested that the combined presence of methyl mercaptan (\(\rm CH_3SH\)), iron sulfide, and nickel sulfide could constitute important components of a “marker” for primitive habitats on Earth and Mars.
6.1.5.4. Hydrogen oxidation and the proton-motive force#
Another significant feature of the methanogenic and acetogenic metabolism is the use of \(\rm H_2\) as the catabolic electron donor. The electron transport chain of many organism is a complex multi-component assembly that moves proteins from inside to the outside of cells as electrons flow from one electron carrier to another. These complex electron chains are characteristic of organotrophs and phototrophs, as well as aerobic lithotrophs that oxidize inorganic compounds other than hydrogen.
The use of hydrogen offers the possibility of a simpler mechanism of a simpler mechanism in which a proton-motive force is generated without the transport of protons. The products of hydrogen oxidation are simply protons and electrons. If the oxidation of hydrogen occurs on the outside of the cell membrane, then protons can accumulate there. A simple electron carrier that spans the membrane can then deliver electrons to the inside. The electrons can then be used on the inside in a proton-consuming reaction to reduce carbon dioxide to methane or acetic acid. The result is a proton-motive force.
This simple mechanism requiring only an extracellular hydrogen-oxidizing enzyme (hydrogenase) and a membrane-bound electron carrier (e.g., iron-sulfur protein) has not yet actually been demonstrated in the methanogens or acetogens. But neither is it excluded as a primordial mechanism or as one that operates today.
6.1.6. Evolution of Photosynthesis#
The evolution of photosynthesis greatly enhanced the capability of the planet to carry out primary production. organisms have evolved to make efficient use of light energy, which has always been available and abundant on the surface fo the planet. Although the evolution of photosynthesis most likely followed the evolution of simpler primary production mechanisms, when it evolved it had a major impact on all life.
Photosynthesis has been found in Bacteria and Eukarya. A remarkable variety of photosynthetic bacterial groups exists. Within the Bacteria, five of the major phyla contain photosynthetic members: Protobacteria, Fimicutes, Chorobi, Choroflexi, and Cyanobacteria. C.B. van Niel proposed (in 1941) the following general reaction for photosynthesis:
where \({\rm H_2A}\) can stand for \({\rm H_2S}\), \({\rm H_2}\), \({\rm H_2O}\), an organic compound, or even ferrous iron (\(\rm Fe^{2+}\)). \({\rm (CH_2O)}\) represents an organic product of the photosynthesis.
Many of the photosynthetic bacteria are restricted to anoxic habitats and ues \({\rm H_2S}\) as a source of electrons. Thus the reaction for these bacteria is:
These phototrophs can often further oxidize the elemental sulfur to produce sulfate anaerobically during photosynthesis:
Cyanobacteria are best known as the first organisms to use water to carry out oxygenic photosynthesis, in which oxygen is produced:
Note that the \({\rm O_2}\) comes from the water and not the carbon dioxide. For this reason, oxygenic photosynthesis is called the “water splitting” reaction. Apart from cyanobacteria, all photosynthetic bacteria carry out photosynthesis anaerobically and oxygen is not produced. This anaerobic process is referred to as anoxygenic photosynthesis.
When did photosynthesis evolve? The availability of suitable substrates is not a constraint because they were all available, as was light. The main constraint has to do with complexity. To generate a proton-motive force photosynthetically requires the addition of chlorophyll, as well as a relatively complex electron transport chain involving the transport of protons across the membrane. This mechanism is more likely to have followed the relatively simple \({\rm H_2}\) chemosynthetic mechanisms.
The time of emergence of different photosynthetic types is unresolved in the geologic record, but available evidence suggests that anoxic phototrophs predated the cyanobacteria. At that time the entire planet was anoxic, since the cyanobacteria had yet to begin to oxygenate the atmosphere and oceans. The evolutionary emergence of the cyanobacteria was the result of a remarkable biochemical innovation, where the development of a light-driven electron transport system that could fix \(\rm CO_2\) with electrons extracted from water, liberating its oxygen. This photosystem, composed of two photosynthetic reaction centers, was likely built upon the machinery of earlier simpler microorganisms that were restricted to anoxic habitats and that used reduced sulfur as a source of electrons.
The time of cyanobacterial emergence has been dated to \(2.1-2.5\ {\rm Ga}\) on the basis of protein sequences, which is consistent with the geochemical estimate for the era of oxygenation of Earth’s atmosphere and oceans (i.e., from the banded iron formations). The evolution of photosynthesis liberated microbial life from dependence on geochemically produced, reduced substrates (e.g., \(\rm H_2S\)), resulting in an explosive increase in biomass on Earth. By using water as a reductant, life was free to fully colonize the planet, now requiring only water, light, carbon dioxide, and organic substrates for growth and division.
The innovation of oxygenic photosynthesis began a remarkable evolutionary progression, culminating in the development of complex animal and planet species. The development of an oxygen-rich atmosphere prepared the biosphere for the emergence of higher life forms. Only by burning reduced carbon with oxygen (via an electron transport chain) could sufficient energy be released to fuel large multicellular organisms. The resultant atmospheric oxygen produced an ozone layer in the statosphere that is strongly absorbent of UV radiation. When the ozone layer became significant, this opened up land to colonization by planets and animals (due to lower UV radiation) who no longer needed water for protection.
6.1.7. Aerobic Metabolism#
Aerobic metabolism is the culmination of metabolic evolution on Earth. This was made possible due to the superior energy yield from using oxygen as an electron acceptor. The microbial world expanded along with multicellular organisms. New kinds of chemolithotrophs: oxidizers of nitrogen, sulfur, and iron compounds evolved and have become an important part of today’s nutrient cycles. The innovations in the electron transport chain that came with photosynthesis allowed aerobes to take advantage of this new source of energy. Indeed, many of the same components of the electron transport chain that evolved for photosynthesis are used in aerobic respiration.
6.1.8. Earth’s Earliest Communities#
If you were to visit the Earth during any average point in its history, the scene would be other-worldly. For most of Earth’s history it was the planet of the microbes. The land would be mostly barren of visible life. Only in shallow freshwater and intertidal marine basins would there be visible accumulation of life, in the form of microbial mat communities. Microbial mats are macroscopically visible microbial ecosystems in which microbes build communities that in may ways are analogous to rain forests. They are layered communities that today develop as carpets, often many centimeters thick, in certain types of shallow aquatic settings.

Fig. 6.2 General structures of microbial mats. The thickness can range from millimeters to several centimeters, and are formed by multiple biofilms of microorganisms embedded in a matrix of exopolysaccharides, in a vertical fashion due to the physical gradients. Image Credit: Prieto-Barajas (2018).#
The structural coherence of the community is provided by extracellular polymers produced by the mat microorganism and by the filamentous forms of some dominant populations. The top layer (i.e., canopy), primarily consists of oxygen-producing bacteria, cyanobacteria. Together with the anoxygenic phototrophs, the cyanobacteria sustain a remarkably diverse undergrowth of other microorganisms. Virtually all major physiological types of microbes are resident in contemporary microbial mats, and they sustain all central biogeochemical cycles, where each element is alternately oxidized and reduced by the metabolism of different microorganisms.
Under the microscope, the activities of these highly organized populations shape the chemical structure of the mat, forming sharp gradients of oxygen, \(\rm pH\), sulfide, and other chemical species (via a metabolic process). High productivity by photo- or chemosynthesis allows each mat to sustain significant biomass on little more than water, sunlight, and available inorganic nutrients.
The most impressive mat communities occur in extreme environments (compared to conditions for multicellular life), since significant biomass accumulation can only occur in the absence of grazing. For example, today’s mats of thermophilic cyanobacteria are common in hot springs worldwide. Mats also thrive in hypersaline lagoons, as well as in intermittently dry intertidal regions. Mats also build calcified stromatolites in warm seas, from desert soil crust, and grow within the frozen rocks of Antarctica as endolithic mats. They even thrive embedded in the ice cover of Antarctic dry valley lakes.
The fossil record suggests that mats were once widely distributed on early Earth. The decline of extensive microbial mat systems in the rock record is generally attributed tot he emergence of multicellular grazers and water planets that ultimate forced them to a much more limited habitat range. The most conspicuous and often enigmatic vestiges of the early microbial biosphere and microbial mats are stromatolites. It is generally accepted (but not always proven) that the sediment trapping or mineral precipitation processes that formed stromatolites were mediated by ancient microbial communities. The existence of fossil stromatolites beginning in the early and middle Archean is consistent with isotopic evidence from autotrophic carbon fixation in the early Archean, and very strongly suggests that photoautotrophy existed.
Today there is increasing recognition that microorganisms are extremely promiscuous. Horizontal gene transfer is not restricted to closely related organisms. Exchange within and between domains is now well documented and increasingly recognized to be a major force in shaping metabolic innovation. We speculate that the high density and close associations among microorganisms in microbial mat provided a superb environment for genetic exchange between populations. If this is true, some of the most significant events in the history of our planet may have occurred in microbial mats. For example, the cyanobacteria may have emerged within this context as a result of melding the photosystems of anoxic phototrophs.
6.2. Limits of Carbon Life on Earth#
The search for extraterrestrial life is intimately linked with our understanding of the distributions, activities, and physiologies of life as we know it, Earth-life. It is important to know the extent of environmental conditions that can support terrestrial organisms as a first-order set of criteria for the identification of extraterrestrial habitats. Even though other life forms may have different biochemistries and origins, the limits of life on Earth may help define the potential for habitability elsewhere.
It is also likely that many of the limits of Earth-life could extend out of the bounds of extreme conditions found on modern-day Earth. This is the case for the bacterium Deinoccus radiodurans, which can tolerate levels of radiation beyond what naturally occurs on Earth. Escherichia coli has an apparent tolerance to hydrostatic pressures that exceed by an order of magnitude the pressures in the deepest ocean trenches.
Since Earth is the only planet that unequivocally supports modern, living ecosystems, it is logical to first look for life elsewhere that resembles Earth-life. Life as we know it requires
either a light or chemical energy source, and other nutrients from its environment (e.g., nitrogen, phosphorus, sulfur, iron, and around 70 other trace elements).
liquid water as a medium (solvent) for both energy transduction and biosynthesis.
Chemical disequilibria are required to fuel the maintenance and growth of organisms. Thus, the search for extraterrestrial life is focused on planets and moons that
currently have (or may have had) liquid water,
have a history of geological and geophysical properties that favor the synthesis of organic compounds and their polymerization, and
provide the energy sources and nutrients needed to sustain life.
However, we are limited by what we currently know about life on this planet. There are two main issues being addressed by astrobiologists:
our incomplete understanding of the physiological diversity of Earth-life, and
our almost complete lack of data about possible alternative biochemistries.
For example, a novel marine photosynthetic microorganism, recently isolated from deep-sea hydrothermal vents, may be utilizing the blackbody radiation from hot sulfides for photosynthesis. In another case, newly discovered microorganism have extended the upper temperature for growth to \(121\ {\rm ^\circ C}\) and lowered the \(\rm pH\) record to below 0. One hyperthermophilic microbe lacks strings of nucleotide bases that are common to all other known organisms in its \(\rm 16S\) rRNA and incidentally, is symbiotic to another archaeal species. Discoveries such as these emphasize how little we know.
6.2.1. Extremophiles and the Limits of Life#
The extreme conditions that limit growth or prove lethal to most organisms may in fact be “Garden of Eden” conditions for other organisms. Extremes of high temperature, high and low \(\rm pH\), high salt concentration, toxic metals, toxic organic chemical compounds, and high levels of radiation kill the overwhelming majority of Earth’s organisms. however, there are organisms from all three domains of life that have adapted to many terrestrial extremes. High temperature, low \(\rm pH\), and high salinity environment are likely to have persisted through Earth history, and these extreme environments are not rare on modern-day Earth.

Fig. 6.3 Representative idealized cross section of Earth’s crust showing the diversity of extreme environments and their approximate location.. Image Credit: Wikipedia:extremophile.#
There are very few natural environments on Earth where life is absent (life is the rule rather than the exception). Microbial life on Earth has proliferated into habitats that span nearly every imaginable environmental variable. Only the very highest temperatures or low availability of water to participate in chemical reactions (e.g., water-ice) render terrestrial environments unsuitable for growth. There are few environments on Earth entirely free from surviving life.
Most discussions of the limits of life focus on the extreme range of single physical or chemical conditions. There does appear to be some absolute maximum temperature and minimum concentration of water that will prevent cellular growth. There are two distinct classes of extreme environmental conditions based on how they affect cells. Extremes in pressure and temperature extend their effect into the cytoplasm. Intracellular biosynthesis, metabolism, and macromolecular structures in extremophiles are adapted to function under such conditions. In contrast, organisms capable of growing in extremes of \(\rm pH\), salinity, irradiation, and in the presence of high levels of toxic metals are adapted to either
maintain intracellular conditions that are typical for non-extremophiles, or
compensate for the extreme condition.
There are some exceptions.
While most acidophiles (“low \(\rm pH\) loving” microbes) maintain an internal \(\rm pH\) near neutrality, Picrophilus torridus grows optimally at \(\rm pH\) of 0.7 and maintains an intracellular \(\rm pH\) value of 4.6.
Among extremely halophilic (“salt loving”) Archaea, some have an absolute requirement for salt and grow best at molar concentrations of \(3.5-4.5\ \rm M\), but can also grow in saturated \(\rm NaCl\) (\(5.2\ \rm M\)). Their intracellular functional and structural components are adapted to high salt concentration and their enzymes require high salt to maintain their active structure.
There are combinations of extreme conditions that apparently prevent cells from growing. For example (so far), no organisms have been characterized that are capable of growing in high salt concentrations at the upper and lower limits of temperature and \(\rm pH\). It is not know whether this combination effect is due to an insurmountable barrier posed by these combinations of extreme conditions, insufficient sample, or lack of assessable habitats with these combinations of extremes.
There are also combinations of extreme conditions that have a synergistic effect on the growth or survival of cells not adapted to either of the specific extreme conditions. This is the case for hydrostatic pressure and temperature, or salt and temperature. Low temperature and high hydrostatic pressures affect cell processes in the same way, with the result that the minimum growth temperature of non-piezophilic (“pressure loving”) microbes is increased with increasing pressure. Similarly, high salt concentrations increase the minimum or decrease the maximum growth temperatures of non-halophilic microbes.
6.2.2. Water, Desiccation, and Life in Non-aqueous Solvents#
The absence of available water and extremes of temperature are the only single variables known to prevent growth and survival of organisms. The other physical and chemical factors that are thought of as extreme conditions (e.g., \(\rm pH\), pressure, radiation, and toxic metals) are life-prohibiting factors for most organisms but not for all. Life has adapted to the entire terrestrial ranges of these variables. however there are some combinations of physical and chemical conditions for which no known organisms have been found to grow. These include environments that have both high salt (\(>30\%\ \rm NaCl\) by weight) and
low temperatures \((<0\ \rm ^\circ C)\), such as in sea-ice inclusions, or
high temperatures \((>90\ \rm ^\circ C)\), known to exist in brine pools beneath the Red and Mediterranean Seas.
Earth-life can be described as a web of aqueous (water-based) chemical reactions. There is a point where the intracellular water activity decrease so far the most cells will die. Desiccation causes DNA to break, lipids to undergo permanent changes and proteins to crystallize, denature, and undergo condensation reactions. Saturated brine pools (\(35\%\) salt, 0.75 water activity) are environments of low water activity that are inhabited by bacteria, Archaea, eukaryotic algae, and brine shrimp.
Organisms that grow or survive in dry environments or in solutions with low water activity match their internal water activity with that of their surroundings. This strategy required evolutionary adaptions of intracellular macromolecules, and of metabolic and biosynthetic processes to operate despite high salt concentrations. Most other microorganisms and eukaryotes deal with desiccation by accumulating compatible organic compounds. Some organisms survive desiccation by forming spores or cysts, while others have mechanisms to repair any damage to their DNA. Both bacteria and eukaryotes have been found to grow in Antarctic rocks that have liquid water for only short thaw periods.
An important issue regarding water and astrobiology is the degree to which water is required by carbon-based life, and whether or not an organic solvent could replace water as the primary solvent. Another issue is the ability of organisms to survive environmental conditions that are outside their limits for growth. These usually involve decreasing the internal water content of the cell, as in the case of bacterial and fungal spores (and some animals such as tardigrades). These issues are important in assessing whether or not carbon-based life could
exist in liquid methane or ethane pools on Titan,
survive the harsh physical conditions that would be encountered during transport from one planet to another, or
survive long periods in completely desiccated state and still retain the ability to grow if water is eventually introduced.
Is it possible for carbon-based life to exist in solvents other than liquid water? many organic solvents including alcohols, phenols, and toluene, are extremely toxic to microorganisms. The degree of antimicrobial action of a solvent depends on its hydrophobicity (“water avoidance”). The more hydrophobic a solvent, the more readily it can accumulate in cellular membranes. The toxicity of the solvents to cells is due to their ability to make the membrane permeable, resulting in the leakage of macromolecules including RNA and proteins.
While organic solvents kill most microorganism, there are some bacteria that can tolerate relatively high concentrations. Two mechanisms have been identified for solvent tolerance:
membranes that limit the diffusion of solvents into the cell, and
specialized mechanisms that remove any solvents that have diffused into the cell.
Another key issue is whether the carbon-based biochemistry can occur in non-aqueous solvents. Certainly, many enzymes function in organic solvents and many organic reactions fundamental to biochemistry can occur in non-aqueous solvents. However, even those enzymes active in organic solvents still have som bound water necessary to maintain their active structure. Water is important in other vital biochemical reactions during metabolism and biosynthesis. It appears that carbon-based life is unlikely to able to adapt to a pure solvent environment unless
it has mechanisms to form water from solvents (e.g., alcohols), or
it can produce all the necessary water de novo (anew) from biochemical reactions.
There exist specific channels in bacterial membranes that function as pores for the transport of water. Named aquaporins, these channels can facilitate rapid water transport during osmotic stress. The presence of specific water-transport pores makes it imaginable that there could be organisms with membranes that are resistant to organic solvents and yet with their specialized aquaporins extract and selectively transport low concentrations of water from the solvent/water mixture. Moreover, up to \(70\%\) of the intracellular water in actively growing Escherichia coli cells is generate by metabolic processes and not derived from the external environment.
6.2.3. Temperature Extremes#
Temperature is a fundamental thermodynamic parameter that affects all biochemical reactions, in particular setting limits on life because temperature and pressure determine whether water is in the liquid phase. Given that liquid water exists in the liquid state, what then is the allowable range of temperatures for life?
Microorganisms have been cultured with growth observed at temperatures as high as \(121\ \rm ^\circ C\) or as low as \(-15\ ^\circ C\). There is even evidence for intact microorganisms with DNA and RNA in hydrothermal vent sulfides at temperatures exceeding \(200\ \rm ^\circ C\). Despite this wide gap in maximum growth temperatures between prokaryotic and eukaryotic cells, eukaryotes share all other extremes of life with bacteria and Archaea, including growth in environments of great acidity, high salt concentration, pressure, and toxic metal concentrations.
Freezing temperatures can kill cells if internal ice crystals are formed. Cells survive freezing if quickly frozen, where viable cells are even preserved for long periods if quickly frozen in liquid nitrogen \((-196\ \rm^\circ C\)). On the other hand, slow freezing or slow thawing of cells favors ice crystal formation that can damage macromolecules and structural polymers leading to death. some cellular adaptations for preventing internal ice crystal formation during slow freezing include increasing intracellular solute concentrations, production of exopolysaccharides, and modification of lipids (and proteins) to increase the fluidity of membranes and mobility of enzymes.
Microorganisms that grow best at temperatures above \(80\ \rm ^\circ C\) are called hyperthermophiles. The maximum growth temperature of cultured hyperthermophiles varies from \(80-121\ \rm ^\circ C\), and the minimum growth temperature varies from \({\sim}40-80\ \rm ^\circ C\), depending on the organism. Hyperthermophiles include bacteria and Archaea, aerobes and anaerobes, and heterotrophs and autotrophs. Some acidophiles, alkalophiles (“high \(\rm pH\)-loving” microbes), and radiation-resistant organisms are also hyperthermophiles.
Hyperthermophiles have protein and lipid structures that are adapted to high temperature. While there are no generalizations that can be made about how enzymes and other proteins are thermally stable, there are some recurrent characteristics.
Protein structures are stabilized at high temperature through amino acid substitutions and most importantly through use of disulfide bonds for structural stabilization.
Heat stable, ether-linked lipids are universal in hyperthermophilic Archaea and in some bacteria.
Fundamental changes in protein and lipid structure compensate for the increased mobility and fluidity at high temperatures.
All hyperthermophiles studied have a reverse gyrase that positively supercoils DNA, which along with cationic proteins increases the thermal stability of the DNA.
Is \(121\ \rm ^\circ C\) the highest possible temperature for growth of life? The chemical properties of water and biological macromolecules to maintain their 3D structure by compensating temperature effects with either higher pressure or with increasing salt concentrations. Important cofactors (e.g., ATP and nicotine-adenine dinucleotide (NAD)) are thermally subject to chemical modifications in order to cope with temperature stress. Hyperthermophiles stabilize ATP and NAD by increasing their rate of synthesis, by extrinsic factors (e.g., high ion concentrations), or by intrinsically more stable replacements. There is also evidence that attachment and biofilm formation on minerals increases the thermal stability of hyperthermophilic Archaea. The upper temperature fo life is still to be determined.
Video Credit: NPS/Hugo Sindelar
Is there a low-temperature limit for life? Microbial activity has been measured at \(-20\ ^\circ C\) in ice, and photosynthesis has been observed in Antarctic cryptoendolithic (“hidden within rock”) lichens at \(-20\ ^\circ C\). Water can remain liquid at temperatures lower than \(-30\ ^\circ C\) in the presence of salts (or other solutes) and at even lower temperatures in combination with soluble organic solvents. Enzyme activity has been measured at \(-100\ ^\circ C\) in a mixture of methanol, ethylene glycol, and water. There is also evidence for the transfer of electrons and enzyme activity at \(-80\ ^\circ C\) in a marine psychorphilic (or cryophilic) bacterium. It is even possible that there may be no lower temperature limit for enzyme activity, nor even for cell growth, if a suitable solvent or solvent mixture is available.
6.2.4. Survival While Traveling through Space#
The ability of microorganisms to survive the most extreme environmental conditions increases the probability that they could survive transport to other planet (and moons) and thereby effect panspermia. Panspermia is an important issue in the context of the “limits” of life since the presence of life in any extraterrestrial setting requires an origin. Two groups of microorganism have received the most attention as possible successful space travelers: spore-forming bacteria and radiation-resistant microorganisms.
Spore formation by bacteria and fungi is usually in response to stress conditions including limit nutrients, drying, and heat-shock. Spores are capable of long-term survival and have been recovered from environmental samples that are more than a million years old. Spores are also known to remain stable against heat and radiation, which allows them to travel on the winds to distant locations, or slowly to sediment down into deep ocean trenches. Some spores can survive more an an hour exposure to dry heat at \(150\ \rm ^\circ C\), although survival is greatly reduced in moist heat. Spores from thermophilic bacteria are more resistant to heat than mesophilic (“moderate temperature loving”) spores.
Any possibility that microorganisms can be transported from one planet to another necessitates that they have the ability to resist the lethal effects of radiation. High-energy radiation and particles (\(\alpha\) and \(\beta\)) damage DNA, which results in cytotoxic and mutagenic effects to the cell. UV radiation is the most abundant form of damaging radiation and probably the most common natural mutagen. Ionizing radiation kills cell in general by causing multiple breaks in DNA, although UV light can also kill cells in other ways which prevent replication. Most organisms protect themselves from damaging radiation with measures such as radiation absorbing pigments and DNA repair mechanisms.
Species |
Phylum |
Dosage (\(\rm Gy\)) that kills |
---|---|---|
Rubrobacter radiotolerans |
11,000 |
|
Deinococcus radiodurans R1 |
Deinoccus-Thermus |
10,000 |
Thermococcus gammatolerans |
Euryarchaeota (Archaea) |
8000 |
Rubrobacter xylanphilus |
Actinobacteria |
5500 |
Chroococcidiopisis species |
Cyanobacteria |
4000 |
Hymanobacter actinoscierus |
Flexibacter-Cytophaga-Bacteroides |
3500 |
Kineococcus radiotolerans |
Actinobacteria |
2000 |
Acinetobacter radioresistens |
\(\gamma\)-Proteobacteria |
2000 |
Kocurea rosea |
Actinobacteria |
2000 |
Methylobacterium radiotolerans |
\(\alpha\)-Proteobacteria |
1000 |
Note
A gray (\(\rm Gy\)) is a unit of absorbed dose of ionizing radiation corresponding to the absorption of \(1\ \rm J/kg\) of absorbing material (\(1\ {\rm Gy} = 100\ {\rm rads}\)). The natural background level on Earth is \({\sim}0.3\ {\rm mGy/yr}\) (at a typical mid-latitude near sea-level). On the surface of Europa the level is almost \(10^{10}\) times higher, enough to kill humans in one minute of exposure.
The most radiation resistant microorganisms include both bacteria and Archaea (see Tab. 6.2). The radiation resistance is many orders of magnitude above what is naturally found anywhere on Earth. Radiation resistance in D. radiodurans is the result of extremely efficient DNA repair mechanisms that with high fidelity can reassemble DNA that has been sheared into multiple pieces. It is believed that thees DNA repair mechanisms evolved in response to DNA damage due to desiccation rather than radiation. T. gammatolerans was found in a submarine hydrothermal vent in the Guaymas Basin off the coast of Baja California at a depth of about \(2600\ \rm m\).
Note
The German cockroach is the most radiation resistant metazoan. The tardigrade in its desiccation-resistant “tun” state can also survive extremely high X-ray exposure.
Microorganisms traveling in space will also be exposed to the interplanetary vacuum of order of \(10^{-14}\ \rm Pa\). Exposure to this level of vacuum causes extreme dehydration of cells such that naked spores can survive for only days. Survival of spores i increased if they are associated with various chemicals (e.g., sugars or embedded in salt crystals). Nicholson et al. (2000) discuss the various stresses that a microbial cell or spore would have to endure to survive interplanetary travel. These include the ability to survive
the process that transports them out of the Earth’s atmosphere (e.g., volcanic eruptions, and bolide impacts),
long periods of transit in the cold of space, and
the entry into a new planetary home.
Spores have been demonstrated to survive the shock conditions of a meteorite impact, UV radiation, and the cold of space. It is clear that panspermia is possible and even probable if bacterial spores become embedded in rocks that get ejected from one planet and eventually enter the atmosphere of another.
There is evidence that microorganisms that are attached to surfaces (e.g., minerals and organic polymers) have enhanced survival to a variety of stress conditions. These biofilms are physically and physiologically complex. The niche within a mature biofilm allows both physiological and genetic transformations of the encapsulated organisms. The immediate environment of cells within a biofilm can affect the delivery of nutrients and the availability of energy sources, which leads to a range of activities. Heterogeneity within biofilms has also been shown to play a role in resistance to environmental stress.
Stress resistance has commonly been attributed to two features of the biofilm microbial communities:
their ability to limit rates of diffusion, and
the presence of multiple physiological states.
Thees physical phenomena are commonly coupled to physiological changes such as the diversity of growth stages, including slow-growing, stationary phase cells.
6.2.5. Extremophiles, Hydrothermal Vents, and Earth’s Earliest Microbes#
Models for early life are based on geological, geochemical, and isotopic evidence of biosignatures in the earliest rocks, inferences from global phylogenetic trees, and the biochemical characteristics of extant organisms. The deep-sea and the sub-seafloor are two of the modeled sites for the earliest microbial ecosystems. These are safe havens from killer impact events, as well as sites of ubiquitous and active geophysical processes, including hydrothermal activity that can generate carbon and chemical energy sources, along with other elements required for life. (see National Geographic: Exploring the Edge of Existence about the discovery of the hydrothermal vents.)
There are models that support the hypothesis that hydrothermal systems were involved in the origin of life and that microorganisms related to extant hydrothermal vent extremophiles were the earliest organisms. The implication is that submarine hydrothermal systems can both generate and support life without the need for oxidants needed for photosynthesis. While these remain hypotheses, there is convincing evidence that microorganisms capable of exploiting the chemical energy sources at vents (particularly \(\rm H_2\)) preceded photosynthesizing microorganisms. Biochemical evidence indicates that the first photosynthetic organisms were anaerobic and coupled the reduction of \(\rm CO_2\) with the oxidation of \(\rm H_2S\) rather than \(\rm H_2O\).
To date (on Earth), we know of two primary types of hydrothermal processes that drive warm- to high-temperature flow in the sub-seafloor.
Ridge-axis, hydrothermal circulation driven by upwelling magma or recently solidified hot rock often producing spectacular hydrothermal chimneys (or “black smokers”). Water-rock reactions commensurate with high-temperature-driven fluid flow result in fluid temperatures up to \(407\ \rm ^\circ C\) and produce distinct chemistries containing high concentrations of metals and low \(\rm pH\).
The exothermic serpentinization of ultramafic rocks, which yields moderate temperature fluids (\(<150\ \rm ^\circ C\)) in comparison to ridge-axis systems. These fluids are highly alkaline (up to \(\rm pH\) 12), and contain elevated levels of volatile compounds (e.g., \(\rm CH_4\) and \(\rm H_2\)) relative to ridge-axis systems.
A third source of hydrothermal activity is tidal heating that occurs when differential gravitational forces from a planet “flex” one of its moons, usually in concert with gravitational effects from other moons. Tidal heating is the best explanation for geysers on Enceladus and is also believed to be important in maintaining a liquid ocean on Europa.
Strong hydrothermally driven fluid circulation is a fundamental physical-chemical process that was likely present on Earth as soon as it had appreciable amounts of liquid water. Hydrothermal activity on the early Earth may have been even more pervasive than today due to higher global heat fluxes (due to much more closely orbiting Moon and geophysical activity). A hallmark feature of hydrothermal systems is dynamic mixing between fluids of varying compositions. These mixing processes occur at multiple spatial and temporal scales, including
the instantaneous process of sulfide mineral precipitation seen at black smokers, and
the modest thermal and chemical gradients in the sub-seafloor.
A vast majority of the mixing occurs within the fractures and pore spaces of rocks, where the fluids interact with and alter the host materials. Hydrothermal systems also experience periodic perturbations linked to the tides, and are intertwined with episodic processes such as earthquake activity, opening and sealing of fractures, and magma intrusion events.
The surface-associated microbiology of present-day hydrothermal vent environments may also be relevant to the origin and early evolution of Earth-life. Abiotic reactions facilitated by mineral surfaces catalyze a number of reactions that may have substituted for early metabolic process. This idea has been expanded to where mineral catalysis could have preceded all enzyme catalysis during the inferred early, non-protein stage in the origin of life.
The aggregated communities found on mineral surfaces may have been important to the early evolution of life in that their diversity could have facilitated a high rate of genetic exchange and over the evolutionary limitations of any single lineage. Hydrothermal systems of the present-day Earth have not changed significantly from those present millions of years ago. Such systems may arbor relict microorganisms and biochemistries that can aid in our understanding of the origin of Earth-life.
6.3. The Evolution and Diversification of Life#
The fossil record conjures up images of dinosaurs, trilobites, and extinct humans. This reflects not only our metazoan-centric bias, but also a visual bias. Starting about \(542\ {\rm Ma}\), macroscopic remains (of animals, traces, shells, and later bones and planets) become quite evident in the fossil record, hence the geological term Phanerozoic, which literally means the eon of “visible life.” These \(542\ {\rm Myr}\) of the fossil record demonstrate that changes have occurred in organisms and have provided compelling evidence for the theory of evolution. See the lectures by Russell Garwood at Univ of Manchester.
The Proterozoic was mainly a microbial world, the Phanerozoic is a world of macroscopic animals and plants. The transition from the Proterozoic to the Phanerozoic marks what my be one of the most significant evolutionary events in the history of life, when many of the modern animal phyla evolved. Highlights of this \(2500\ {\rm Myr}\) record include
the rise to dominance of cyanobacteria in shallow marine environments,
the early evolution and diversification of eukaryotes,
the first appearance of multicellular algae,
the appearance and their subsequent diversification (the Cambrian explosion),
the first land plants and their subsequent diversification,
the appearance and dominance of intelligent life.
In addition to these biologic changes, the Earth’s crust continued to be dynamic with active seafloor spreading and plate tectonics; the atmosphere changed from an anoxic to an oxygenic atmosphere; and the oceans responded to theses and other biospheric, lithospheric, and atmospheric changes.
For astrobiology, an understanding of the evolution and diversification of life on earth is critical. The Earth is the most active of all the planets in the solar System and continues to evolve. Reading the fossil and rock records provides valuable insight into life’s evolution and diversification. Although the search for extant life elsewhere in the Universe is of tremendous importance, such a search would focus only on the very narrow window of the present time. Searches should also be made at extraterrestrial sites for fossil life, since such a search takes full advantage of the immense spans of time involved with a planet’s history.
6.3.1. The Proterozoic#
The Proterozoic spans almost \(2\ {\rm Gyr}\) recording the further evolution and diversification of already established bacteria and eukaryotes. This long record documents important evolutionary changes that shaped the biosphere on its way to a planet dominated by planets and animals.
The term Proterozoic was coined from combining the Greek words protero (meaning anterior or earliest) and zoic (meaning life or animals). Historically, rocks older than the Cambrian were though to lack fossils. However, indications of fossils were discovered as the sedimentary rocks found below the Cambrian (layer) received more attention. This suggested the possibility of a longer history of life than previously thought. Based on a variety of studies, it became established that the Proterozoic contained significant remains of life, but it was dominated by microorganisms.
All three domains of the tree of life (Bacteria, Archaea, and Eukarya) were present by the beginning of the Proterozoic. This included the establishment of anaerobic heterotrophs, lithotrophs, and photoautotrophs as well as variations on these metabolic pathways. During the early Proterozoic cyanobacteria had already evolved oxygenic photosynthesis. With the rise of an oxygenic atmosphere, microbes using oxygen greatly diversified, where aerobic metabolism was essential for the evolution of eukaryotes with the already established Eukarya domain. It was during the Proterozoic that three of the four major eukaryotic groups evolved (algae, fungi, and animals), where plants did not appear until the early Paleozoic.
6.3.1.1. Types of Proterozoic fossils#
Proterozoic fossils consist fo the following major types: microfossils, stromatolites, macroscopic carbonized compressions, soft-bodied impressions, trace fossils, and mineralized hard parts.
Microfossils are the preserved remains of microbes. There are two primary methods of study: acid residues and petrographic thin sections. Both rely on light microscopy for examination (i.e., techniques using a microscope).
To prepare a petrographic thin section, a thin sliver of rock is cut from the sample and epoxied to a glass microscope slide. The sliver is further thinned by grinding to a thickness (\({\sim}30-50\ {\rm \mu m}\)) that allows the transmission of light. Microbes are preserved in situ within the layers of sedimentary rock.
For acid residues, rock samples dissolved in acid are examined for insoluble residues under a microscope. Organic walled remains in the residues are commonly called acritarchs.
Stromatolites are laminated structures produced by the sediment trapping, binding, and/or mineral precipitation of microbes (principally cyanobacteria). They are abundant and diverse in Proterozoic carbonate rocks.
Carbonaceous compressions or carbonaceous films are compressed, chemically resistant, organic material visible to the naked eye. Because of their macroscopic size and regular shape, many are considered to be eukaryotic fossils.
Soft-bodied organisms can be preserved as impressions on bedding planes. For the Proterozoic, the most famous of these fossils is the Ediacaran biota.
Trace fossils are the tangible evidence of the activity of an organism (e.g., burrowing). There are numerous reports of trace fossils older than \(600\ {\rm Ma}\), but these reports have not held up to scrutiny.
Mineralized skeletons (hard parts) by eukaryotes very late in the Proterozoic gave rise to the rich, macroscopic fossil record that characterized the Phanerozoic.
6.3.1.2. Prokaryotes#
Archaea and Bacteria are two distinct groups that comprise prokaryote microorganisms. Archaea differ from Bacteria in cell wall composition, the structure and composition of membranes, and other differences. There is no direct, body fossil evidence for Archaea in the Proterozoic or earlier. However, there is indirect evidence based on sediments with highly depleted \(\delta^{13}{\rm C_{org}}\) that are indicative of methanogens in the Late Archean.
The record for Bacteria in the Proterozoic is good an is actually better than in the Phanerozoic. Although the Proterozoic microfossil record is dominated by cyanobacteria, this does not necessarily mean that they dominated the biosphere. Rather, it could simply be that cyanobacteria were more readily preserved than other bacteria. In the Proterozoic, microbial mats were likely geochemical sites for early silicification (chert, or microcrystalline quartz, formation), which favored the preservation of cyanobacteria. In addition, the chemistry and structure of cyanobacterial sheaths and cell walls probably favored their silica permineralization over other prokaryotes.
For smaller (\(<2\ {\rm \mu m}\)) spheroidal and filamentous microbial fossils, bacterial taxonomic assignments other than cyanobacteria are possible even though the small size and simple morphology are shared by many groups of bacteria. Sometimes the microbial fossil has a distinctive morphology or biomarker studies can be used to aid in the determination of bacterial taxonomic assignments.
6.3.1.3. Stromatolites#
The most obvious fossils from the Proterozoic are the centimeter to millimeter sized stromatolites. Many Proterozoic shallow, marine carbonate units contain stromatolites of a variety of shapes. Shapes include wavy-laminated stratiform structures, domes, columns, branched columns, cylindrical cones, and oncoids (i.e., laminae that almost or entirely enclose a central core).
The sizes of Proterozoic stromatolites span six orders of magnitude, ranging from columns several millimeter in diameter to domes over \(100\ {\rm m}\) across. As highly variable as this might seem, one of the hallmarks is that within a given bed (along the same horizon) the shape and size of the stromatolites are often narrowly restricted. This gives the sense that there is a morphological theme to the stromatolites in the bed. The morphological theme of
relatively narrow size range,
the sam or nearly the same shape, and
a distinctive microstructure
has resulted in the taxonomic treatment of a number of stromatolites. These distinctive stromatolites are even treated like fossil species. Although this practice is controversial , a number of stromatolite taxa can be easily recognized and used as index fossils for determining the age of the strata. About 1200 species-level taxa have been treated taxonomically, of which 90% of these taxa are from the Proterozoic.
Below you can find some 3D models of stromatolites – please do take the time to look at these (you may want to close one before opening the next so only one is open at any time). They are actually quite common if you do geology fieldwork, and are the first macroscopic evidence of life on earth!
Fossil specimen of a stromatolite from the Silurian of Herkimer County, New York. Specimen is on display at the Museum of the Earth, Ithaca, New York. Model by Emily Hauf.
Fossil specimen of the stromatolite Collenia versiformis from the Proterozoic of Montana. Specimen is from the Cornell University Paleobotanical Collection (CUPC), Ithaca, New York.
Fossil specimen of the stromatolite Chlorellopsis coloniata from the Eocene of Wyoming. Specimen is from the Cornell University Paleobotanical Collection (CUPC), Ithaca, New York. Model by Emily Hauf.
6.3.1.4. Acritarchs#
The term acritarch was created to accommodate organic-walled microfossils of uncertain taxonomic affinity that were found in acid-resistant residues of shale and siltstone. Acritarchs come in a variety of shapes, with or without ornamentation (some have spines or elaborate processes), and sizes (a few \(\rm \mu m\) to several \(\rm mm\)). They are usually considered to be cysts, spores, or vegetative unicells of algal phytoplankters (microscopic algae that live int he upper part of water columns), although other origins are possible.
Possibly the oldest acritarchs (sphaeromorphs around \(48\ {\rm \mu m}\) in diameter) come from \({\sim}2.1\ {\rm Ga}\) shale in Siberia, suggesting the establishment of eukaryotic phytoplankton by this time. Acanthomorphs (complex acritarchs with spines or other projections) are more confidently interpreted as planktonic eukaryotes, and are known from strata \({\sim}1.5\ {\rm Ga}\) in Australia. Acritarchs are the most abundant eukaryotic fossils found in the Mesoproterozoic and Neoproterozoic.
6.3.1.5. Other algae, amoebae, and fungi#
Some microfossils that are morphologically distinctive can be attributed to specific eukaryotic clades (and hence are not called acritarchs). Microscopic red algae (Bangiomorpha) are found in \(1.2\ {\rm Ga}\) rocks of Arctic Canada. Chrysophytes (also known as golden algae) are heterokonts, which are a group of eukaryotes that includes diatoms, brown algae, and water molds that underwent a secondary symbiosis involving a red algae and possesses two different flagella.
Calcification in algae appears to have occured earlier than in animals. Thin, sheet-like calcareous structures have been found in the Kingston Peak Formation (\(700-750\ {\rm Ma}\)) in California. It is not until the latest Neoproterozoic that calcareous algae and calcified cyanobacteria became more abundant and diverse. Vase-shape microfossils common from several late Neoproterozoic localities are considered to be testate amoebae (protozoans) based on their strong similarity with living examples.
The fossil record of fungi is known almost exclusively from microscopic remains. Based on molecular clock analyses, fungi are generally considered to have originated in the Proterozoic. One microfossil from \(1.43\ {\rm Ma}\) rocks (Tappania) might also be a fungus.
6.3.1.6. Carbonaceous compressions#
Millimeter and larger-sized carbonaceous compressions from the Proterozoic constitute an interesting type of fossil. Based on their macroscopic size and distinctive shapes they are considered to be multicellular eukaryotes. Disks, ellipses, ribbons, sausage-like shapes, leaf-like shapes, and irregular shapes are some of the morphologies found. These shapes continue into the Mesoproterozoic, however, the diversity and complexity increases in the Neoproterozoic. Most are considered to be formed by algae, although some ring-shaped forms from \(800-850\ {\rm Ma}\) rocks have been interpreted as worms or worm-like metazoans.
6.3.1.7. Animals#
The fossil record of Proterozoic animals is controversial, but the most confident remains consist of an assemblage of soft-bodied animals (the Ediacaran biota) from late Neoproterozic strata worldwide (except Antarctica). The ages of these fossils range from \(540-565\ {rm Ma}\), where the Ediacarans comprise a wide variety of soft-bodied marine animals with some forms suggestive of jellyfish, worms, and sea pens (see lectures from Thomas Holtz at UMD). Some fossils do not resemble any living counterparts. Because of the unique morphology of many, the animal affinities have been questioned and it has been proposed that these represent an extinct Kingdom.
Animal trace fossils are also known from Ediacaran-aged rocks. Most traces are millimeter sized horizontal trails, bu some show shallow sediment penetration (i.e., burrowing). Pre-Ediacaran trace fossils are more controversial, where the controversy often centers on the unexpected great age. Curiously, some of these structures would likely be accepted as trace fossils if they were found in younger (Phanerozoic) rocks.
One report consists of millimeter diameter, sinuous traces from strata in India that was thought to be \(1.1\ {\rm Ga}\). This report received considerable attention, in large part because the structures looked like animal traces, but were almost \(500\ {\rm Myr}\) older than the previously accepted oldest animal traces. Recently, new radiometric age dates on the rocks yielded an even older age: \(1.6\ {\rm Ga}\). It is likely that these are not animal trace fossils.
Another report describes possible trace-like fossils from \(>1.2\ {\rm Ga}\) rocks in Western Australia. It is not clear if theses structures (a) were produced by animals, (b) represent some extinct lineage independent of the metazoans, or (c) had an origin independent of organisms (i.e., abiogenic).
The evolution of the Eukarya (as seen from the fossil record) was one of the increased morphological complexity. This complexity included
an increase in intricacy of cells and their coverings,
an increase in the number of cell types,
the evolution of multicellularity,
the development of a tissue grade of organization, and
the formation of organs.
Much of this was accompanied by an increase in size to macroscopic proportions. The development of eukaryotes may have been influenced by oxygen in the atmosphere. Oxygen levels rose significantly at least twice in the Mesoproterozoic and Neoproterozoic, once at about \(1.3\ {\rm Ga}\), and again in the late Neoproterozoic. The close of the Proterozoic saw an end to the dominance of prokaryotes in the fossil record. the biosphere was beginning to be taken over by macroscopic eukaryotes. The pathways taken by evolution would profoundly change as animals and plants expanded in the biosphere.
6.3.2. The Phanerozoic#
The Phanerozoic is an eon characterized by macroscopic animals and planets, which has lasted \(542\ {\rm Myr}\). However, the microbial world of the Proterozoic has not really disappeared, where it has been overshadowed by these large forms of multicellular life. The fossil record of the Phanerozoic is understood much better that the Proterozoic, not only because of its richness, but also from the fact that many more paleontologists study its plants and animals compared to study of the Proterozoic (i.e., the fossils of the Proterozoic are much more difficult to study). The biostratigraphic and absolute time control on the fossil record is also excellent. Hence, patterns of increased diversification of a group of organisms (i.e., radiations) and extinctions of fossil groups are quite well defined and contribute significantly to our understanding of evolution on planet Earth.
6.3.2.1. The Cambrian “Explosion”#
The appearance of organisms in the fossil record with hard parts (e.g., shells) signals the transition from the Proterozoic to the Phanerozoic. The first \(54\ {\rm Myr}\) period of the Phanerozoic (i.e., the Cambrian Period) marks the most important interval in the evolution of life on Earth. It is during this time that all the major modern animal phyla either appear or greatly diversify. Known as the Cambrian “explosion,” the nature and cause of this seemingly abrupt appearance of animals has been (and continues to be) the subject of much debate. The question is: was this a real event (i.e., a sudden explosion in lifeforms), or was it due to the nature of the fossil record, which can now much more readily be preserved through their evolved hard parts?
The Neoproterozoic-Cambrian (sedimentary) boundary is available for study in many areas around the world. Studies have shown a general pattern with regard to the order in which the animal fossils appear in the strata. Below the boundary, low diversity faunas of both body and trace fossils are abundant, while above one finds diverse metazoan assemblages dominated by arthropods (e.g., trilobites). Either fortunately (or due to the nature of the Cambrian environment) there is a disproportionately large number of exceptionally preserved fossil deposits in which organisms (with and without hard parts) have been preserved. The two most important are the Early Cambrian Chengjiang biota (in China) and the Middle Cambrian Burgess Shale biota (in Canada).
Note
A fauna (pl. faunae) is a collection of associated animals, and a flora (pl. florae) is similarly for plants.
The fossil record indicates a short interval of only \({\sim}30\ {\rm Myr}\) from the time of the Ediacaran fauna to the early Cambrian, in which most modern phyla evolved. Studies of molecular clock data from living organisms propose a very much earlier appearance for metazoans (as much as \(1.5\ {\rm Ga}\)). The fossil record suggests that only five of the modern animal phyla had appeared before the Cambrian:
sponges,
stem-group cnidarians (e.g., polyps),
anthozoans (e.g., coral),
stem-group lophotrochozoans (e.g., tubes), and
possibly platyhemlinthes (e.g., flatworms).
During a period in the Early Cambrian (maybe as short as \(10\ {\rm Myr}\)), the other major phyla appeared, including ctenophores, priapulids, nematodes, lobopodians, arthopods, moluscs, halkieriids, annelids, brachiopods, hemichordates, echinoderms, cephalochordates, and chordates (which include all vertebrates).
One of the challenges in interpreting the extent of the Cambrian evolutionary explosion is the degree to which the early Phanerozoic faunas were related to the Edicacaran faunas. At least some of the organisms would appear to have similarities with modern phyla. The most significant of these are the sponges, anthozoan cnidarians (corals), and stem-group triploblasts. One of the features that characterizes the Cambrian explosion is the evolution of biomineralization, which is the ability to produce minerals, (usually based on \(\rm Ca\), but also \(\rm Si\) and \(\rm P\)) through metabolic processes that results in shells or other hard parts.
The Cnidarians are not very common in Cambrian strata. The Chengjiang and Burgess Shale faunas indicate that arthropods and sponges were the dominant groups in the Early to Middle Cambrian seas. Following their appearance in the late Proterozoic, sponges diversified greatly in the Cambrian. The Chengjiang fauna play a major role in the formation of the first Cambrian reefs.
Arthropods comprise \({\sim}45\%\) of the Chengjiang and Burgess Shale faunas. Studies suggest that arthropods occupied a range of ecological niches, having evolved morphological adaptions linked to defense and feeding strategies. The geologically simultaneous appearance in a number of phyla of biomineralization argues for strong survival advantages for these hard, outer body coverings. This may have been for protection in response to the evolution of predator/prey relationships. The top-line predator was Anomalocaris (“abnormal shrimp”), which is a form that links the more primitive lobopods with the more advanced arthropods (i.e., crustaceans and trilobites).
As to what triggered the Cambrian explosion, the mechanisms are still obscure. Studies of isotopic and chemical indicators (principally \(\delta^{13}{\rm C}\), \(^{87}{\rm Sr}/^{86}{\rm Sr}\), and phosphogenesis) indicate that significant changes were occurring in ocean chemistry an circulation. The extent to which these affected or stimulated the evolutionary radiations (or rather are a reflection of it) is unclear. The Proterozoic-Phanerozoic transition is characterized by extensive phosphogenesis, deposition of black shales, and extreme negative shifts in \(\delta^{13}{\rm C}\). The transition is generally considered to be the result of changes in global tectonics, ocean circulation, and/or nutrient supply.
It is also possible that the chemical changes are a result of the Cambrian explosion arising from the development of more complex food webs. Analysis of the Burgess Shale community has indicated the presence of feeding habits analogous to those existing in present-day marine ecosystems. Marine primary productivity is likely to have increased significantly with a major diversification of phytoplankton int the Cambrian. Accompanying this (and perhaps driving it) was the evolution of zooplankton, which may have been a key innovation that led to the evolution of larger animals. Zooplankton fecal pellets may have played a role in oxygenation f the sea floor, as well as increasing the availability of nutrient-rich organic compounds to the seafloor biota.
Ecological factors likely drove the evolutionary radiations of metazoans and phytoplankton. In particular, the appearance of herbivores and carnivorous predators that engendered evolutionary “arms races” between predators and prey, as well as the evolution of a range of new feeding strategies. These include carnivorous behavior, scavenging, and filter feeding in the ocean. Poor regulation of genes controlling development would have resulted in significant morphological diversification over short time periods. It is suggested that poor regulation of homeotic genes in trilobites during the Early Cambrian may have been a factor in driving evolutionary radiations in trilobites.
6.3.2.2. Bacteria and Plants on Land#
There earliest evidence for colonization of the land comes form \(1.2\ {\rm Ga}\) rocks in central Arizona, where microfossils represent the remains of microbial communities on land. These have morphologies and sizes suggestive of bacteria (including cyanobacteria), and are though to be have been living at or close to the land surface. Evidence for biological activity in these rocks is supported by \(^{13}{\rm C}\) depletion, caused by \(\rm CO_2\) respiration into groundwater by terrestrial (i.e., land-based as opposed to marine) organisms, which may have also contributed to weathering of the rocks.
The presence of spore-like structures in Middle Cambrian shales in Tennessee and the Grand Canyon may be the first indication of the presence of land plants. These simple spheres are though to have derived from very primitive land plants, and were probably closest to simply bryophytes (mosslike plants). The large morphological diversity of theses structures indicates a relatively diverse flora on land at this time. This would produce significant consequences for atmospheric evolution. The presence of a Cambrian terrestrial flora living by photosynthesis may have played a significant role in drawdown of atmospheric \(\rm CO_2\). It has been suggested that this might even have played a pare in the Cambrian explosion, where carbon runoff affected the trophic structure of shallow water ecosystems.
6.3.2.3. Land Invertebrates#
The fossil record indicates that the first metazoans to colonize the land were arthropods. Arthropod tracks from \({\sim}500\ {\rm Ma}\) sandstones in Ontario (Canada) have been interpreted as having been made out of the water on land. These arthropods were likely to have been amphibious, and would have faced immense physiological problems in making the transition from an aqueous to a dry environment. The much greater diurnal changes in the atmosphere than in the aquatic environment presented problems of potential desiccation. A major limiting factor in the transition to air-breathing was the problem of \(\rm CO_2\) excretion in air. The success of arthropods in early land colonization lay in their tough exoskeleton, pre-adapted to a life on land.
Other evidence for animal activity on land is Late Ordovician (\({\sim}450\ {\rm Ma}\)) burrows in fossil soils in Pennsylvania that may have been made by millipedes. These small arthropods may have overcome high temperatures and potential desiccation by burrowing in the soil. Fossilized fecal pellets from Later Silurian deposits (\({\sim}410\ {\rm Ma}\)) deposits in Sweden that contain fungal hyphae indicating the existence of fungivorous microarthropods (e.g., mites or millipedes). This implies the presence of a decomposer niche, with the arthropods playing an important role in the establishment of soils through reworking and increasing nitrate and phosphate levels. This would have provided a necessary habitat to allow subsequent colonization by vascular plants, which are those with a system of tissues for circulating water and nutrients.
The presence of book lungs similar to those in modern spiders provides firm evidence that animals had achieved a major step in living on land: respiring in a gaseous atmosphere. Slightly younger Middle Devonian remains from Gilboa in New York State have yielded the earliest known spider, Attercopus fimbriunguis. Like modern spiders it is equipped with a spinneret, providing evidence that even the earliest spiders were able to spin webs. It was also endowed with fangs and a poison gland. Like the arthropods, these spiders were mainly predators and were probably feeding on abundant mites and millipedes.
There is little evidence for herbivorous arthropods in the fossil record until well into the Carboniferous Period (\({\sim}350\ {\rm Ma}\)). Eating plants may only have become established when animals had evolved appropriate enzymes and digesting symbiotic bacteria in their gut. Before this adaptation, the vascular plants could have been toxic to early terrestrial animals.
6.3.2.4. Land Vertebrates#
Vertebrate colonization of the land occurred some time after the arthropod invasion. The oldest tetrapod (four-legged animal) evidence is incomplete remains of Elginerpeton from the Late Devonian Scat Craig deposit (\({\sim}370\ {\rm Ma}\)) (near Elgin, Scotland). This amphibian was very fish-like in its anatomy, and presumably in its behavior. The most complete of the early tetrapods are Ichthyostega and Acanthostega form the Late Devonian of East Greenland. See the Lecture Notes from Thomas Holtz @ UMD.
Detailed work on their limbs has revealed that Ichthyostega had seven digits on the feet, whereas Acanthostega had eight digits. It has been suggested that the appearance of digits was probably an adaptation for swimming rather than for walking on land. This casts doubt on the terrestrial ability of these tetrapods. Studies of the braincase, limb skeletons, and gill arches indicate that these early amphibians were little more than fish with slightly modified skull patterns, and digits rather than fins on the ends of their limbs. They were largely aquatic, possibly venturing onto land for short periods.
The fish-tetrapod transition was complex, involving large morphological and physiological changes. These include
the development of air breathing,
the ability to walk on land,
the ability to hear in air,
the ability to retain moisture, and
the ability to reproduce out of water.
It is likely that these steps occurred one at a time and not all at once.
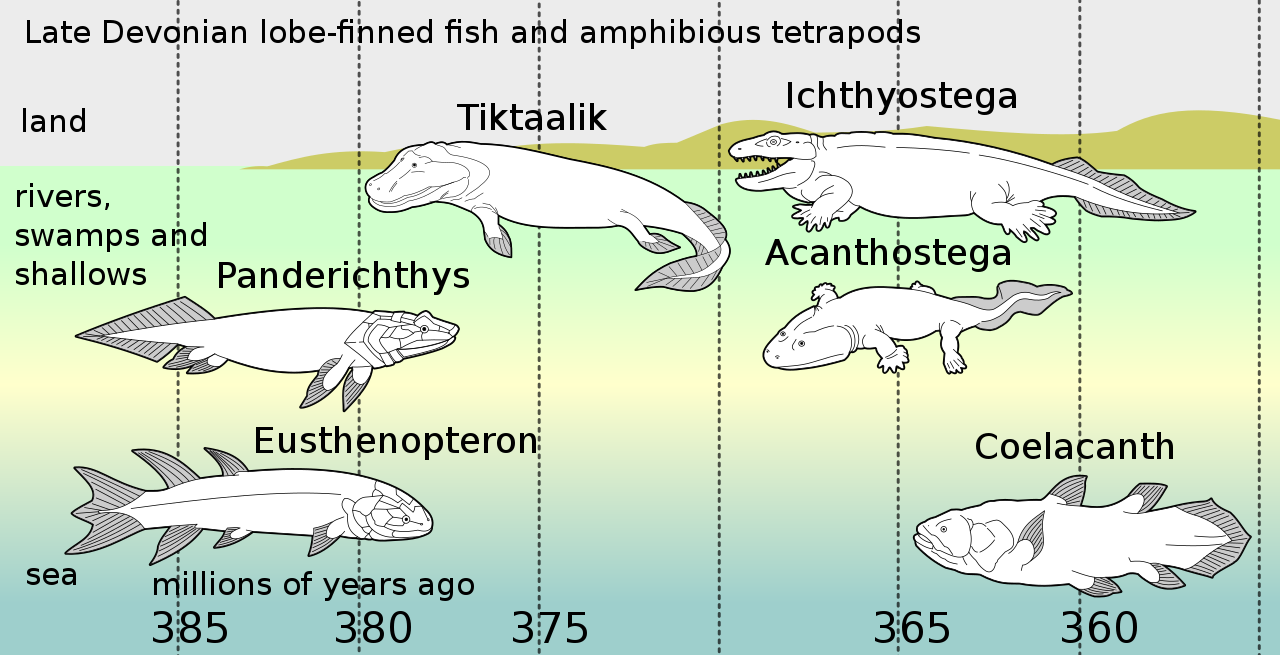
Fig. 6.4 Late Devonian lobe-finned fish and amphibious tetrapods. Image Credit: Wikipedia:evolution of tetrapods.#
The first tetrapods did not rush out of the water, but emerged fully equipped and pre-adapted for a life on land. The earliest tetrapod to have been adapted for walking on land was Pederpes from the early Carboniferous (\({\sim}300\ {\rm Ma}\)) of Scotland. While the presence of a lateral line indicates Pederpes was partly aquatic, the structure of its foot suggest that is was also adapted for walking on land.
The selection pressure that “drove” vertebrates onto land may well have been to find new prey. Panderichthyid fish (from which tetrapods probably evolved) are though to have been predators, possessing very large heads relative to their body size, large fangs, and a wide gape. Their well-developed hands and feet (while not initially evolved for locomotion on land) were pre-adapted for supporting their body weight out of water. The positioning of their eyes on top of the skull meant that they were perfectly suited to viewing a new, terrestrial world.
The first tetrapods possessed a long fish-like tail, little changed from the panderichtyid fish tail. Even after the more advanced amphibians developed improved limb girdles that allowed them to walk freely on land, the tail remained as the important propulsive device for aquatic forays. The first amphibians retained a body covered by fish-like scales. It has been suggested that a scale-covered belly would have provided important protection when dragging themselves across the ground and it would have assisted in preventing desiccation.
6.3.2.5. The Aerial Niche#
It was not until the middle Carboniferous (\({\sim}325\ {\rm Ma}\)) that the first organisms occupied the aerial niche. Arthropods again led the way, this time in form of flying insects. the first insects were flightless and evolved in the Early Devonian, but then insects are absent from the fossil record for \(55\ {\rm Myr}\). During this time, the first flying insects must have evolved, as ten orders of flying insects suddenly appear in the fossil record at the Middle/Early Carboniferous boundary (\({\sim}335\ {\rm Ma}\)). The evolution of the largest known flying insects early in the group’s evolutionary history were dragonflies with wingspans up to \(0.7\ {\rm m}\). This may have been in part due to the high atmospheric levels of \(\rm CO_2\) and \(\rm O_2\) at this time, which resulted in higher atmospheric pressures capable of supporting such large bodies.
6.3.2.6. Marine Faunae#
The fossil record of the Phanerozoic is dominated by marine organisms, mainly invertebrates. This is due to the enhanced chances in marine settings of burial in sediments after death (i.e., easier for such life to be recorded). Moreover, for the Paleozoic, the fossil record indicates that most organisms were aquatic. Studies of fauna during the Paleozoic comprises of the Cambrian fauna (dominated by trilobites) and the post-Cambrian fauna (i.e., Paleozoic fauna).
The Cambrian evolutionary radiation was characterized by the origin and diversification of basic body plans and diversification of basic body plans at the phylum and class levels. There was a three- to four-fold increase of global richness of marine families during the Ordovician compared to the Cambrian. The dominant classes to radiate were primarily immobile, suspension-feeding species (e.g.e, articulate brachiopods, crinoids, cephalopods, gastropods, and bivalves).
Many of these groups were associated with reef systems that became dominant in low latitude from mid-Ordovician times onward. On a global scale, Paleozoic fauna went into decline during the Devonian. Fish diversified greatly during hte mid-Paleozoic times, particularly sharks, osteichthyans, acanthodians, and placoderms.
Shallow seafloor communities experienced the Mesozoic “marine revolution” from \(251-65\ {\rm Ma}\). This was characterized by a diversification in durophagous predators (shell crushers) and by an intensification of grazing by both vertebrates and invertebrates. During the Jurassic, some sharks, rays, and crustaceans evolved the habit , while during the Cretaceous it appeared in a number of groups gastropods.
What drove this Mesozoic marine revolution? Evolutionary arms races between predators and prey contributed, but nonbiological factors may also have been significant. Correlations appear between the intervals of climatic warming, marine transgression (sea level rising and covering land), and high productivity. During the late Mesozoic, periods of massive submarine volcanism contributed enhanced nutrients to the marine environment. In addition to the elevated temperatures, the levels of productivity increases and thus enabled the evolution of lifestyles that utilized higher energy levels (e.g., more calcification and higher metabolic rates supporting more active organisms). The two principal phytoplanktonic algae in the oceans today (the diatoms and coccolithophorids) also appear in the Mesozoic.
Biotic recovery during the Cenozoic was variable after the Cretaceous-Tertiary (K-T) mass extinction event eliminated \(60-75\%\) of all species \(65\ {\rm Ma}\). The extinction removed a sizeable part of the food chain, which resulted in profound and extended reorganization of nutrient and biogemochemical cycling in the oceans. Re-establishing pre-extincition levels of morphological, taxonomic, and ecological diversity occurred by a series of discrete radiations.
While some groups (e.g., ammonites and marine reptiles) became extinct, others (e.g., echinoids) saw little change in diversity across the boundary, but later underwent major changes. These include the evolution of the clypeasteroids (sand dollars), which have diversified throughout the Cenozoic. The numbers of microplankton never returned to those of the Mesozoic. Higher diversity occurred during the warmer phases, notably the Paleocene-Eocene and the Miocene.
When compared with pre-Cenozoic times, the marine biota experienced great increases in diversity through the Cenozoic after recovering from the K-T event. The Cenozoic marine biota can therefore be regarded as essentially a continuation of the Modern fauna that first appeared during the early Mesozoic. The most significant chage in composition of the marine fauna, was the evolution of marine mammals during the Eocene and their subsequent diversification.
6.3.2.7. Terrestrial Florae#
During Cambrian-Ordovician times (\({\sim}542-450\ {\rm Ma}\)), atmospheric \(\rm CO_2\) levels may have been up to 18 times higher than present-day levels. This played an important role in soil formation since more acidic rain increased chemical weathering of rocks, so helping soil formation and providing an environment in which vascular plants could grow and diversify. Moreover, \(\rm CO_2\)-enrichment favored photosynthetically active organisms that promoted the decomposition and release of inorganic nutrients. however, the very high \(\rm CO_2\) levels were not beneficial for terrestrial plants as they contributed to very high global temperatures. Only when global temperatures began to decline did vascular plants evolve and diversify.
Over the next \(50 \rm Myr\), terrestrial flora underwent its greatest diversification, evolving from small vascular plants to dense vegetation with trees over \(35\ \rm m\) tall. During the early period of vascular plant diversification in Early-Middle Devonian times, \(\rm CO_2\) levels were possibly \(8-9\) times higher than now. This was one of the most active periods in the Phanerozoic of plate movement and dramatic global climate change, and probably contributed significantly to floral diversification.
During the Carboniferous, global climate changed from warm, humid, and ice-free to cooler and drier with a glaciation at high latitudes in the Souther Hemisphere. This caused a drop in sea level of \(100-200\ \rm m\) and aridity in high latitudes. But in a narrow equatorial belt, rain all year meant that vegetation accumulated in lowland swamps and led to the formation of the great coal deposits of North America and Europe. The continental blocks of Gondwana and Laurasia joined to form the supercontinent of Pangea (late in the Early Carboniferous).
From the Late Devonian-Carboniferous (\({\sim}300-370\ {\rm Ma}\)), \(\rm CO_2\) levels are though to have plummeted from \(3600\) to \(300\ \rm ppm\), contributing significantly to global cooling. \(\rm CO_2\) levels are estimated by using the density of stomata (pores) on fossil leaves (i.e., the greater the density, the lower the \(\rm CO_2\) levels). During the diversification of vascular plants, their roots contributed to increasing weather, and consequent drawdown of atmospheric \(\rm CO_2\). Some of the \(\rm CO_2\) became dissolved bicarbonate and was transported to the oceans by rivers, while some was locked away as coal in the large accumulations of organic material.
During the Devonian-Carboniferous, plants not only grew larger, but developed more sophisticated reproductive structures, water transport systems, and root and leaf architecture. Leaves first appeared as very small structures during the Early Devonian, then became larger and broader by the end of the Devonian. This was presumably in response to the reduction in atmospheric \(\rm CO_2\), as more leaf area is required to accommodate more stomata. The evolution of seeds during the Devonian revolutionized the plant cycle by freeing plants from the need for external water for sexual reproduction (i.e., plants could grow farther away from bodies of water).
The first trees appeared during the mid-Devonian (\({\sim}380\ {\rm Ma}\)). For the next \(80\ {\rm Myr}\), forests of spore-producing trees, plus two groups of early seed-producing trees dominated the landscape. The spore-producing trees where lycopsids (e.g., Lepidodendron), which represented two-thirds of early forests, and sphensopsids (e.g., Calamites), which flourished in swampy conditions. Other significant elements of the flora were ferns, progymnosperms (ancestor of all seed plants and conifers), pteridosperms (seed ferns), and Cordaites (a common Carboniferous plant).
During the last \(65\ {\rm Myr}\), continental planets have moved into their present configurations that produced mountain ranges (e.g., Alps, Himalayas, and Rockies). Global climate has changed from a very warm greenhouse world in the early Cenozoic to an icehouse world in recent times. Early Paleocene to Middle Eocene (\(65-45\ {\rm Ma}\)), was a warm period in Earth’s history. For example, the deep oceans reached \(9-12\ {\rm ^\circ C}\) higher than present-day levels and annual sea surface temperatures around Antarctica were \(15-17\ {\rm ^\circ C}\), while mean diurnal temperatures at \(45^\circ\) latitude reached about \(30\ {\rm ^\circ C}\). This was due to a number of factors:
changing continental configurations affecting ocean currents and global climate,
the Pacific Ocean enlarging and taking warmer water to high latitudes,
increased mantle degassing,
increased volcanic activity,
methane-induced polar warming, and
a period of global carbon imbalance (i.e., more \(\rm CO_2\) production than burial).
At this time, the poles were ice-free, precipitation occurred more inland, and temperature gradients from the equator to the poles were much smaller than now.
During these profound environmental changes: angiosperms diversified, forests shrank, and grasslands expanded. Forests and woodlands of angiosperm trees extended from pole to pole. Continents were dominated by an extensive tropical everwet biome that extended to relatively high latitudes. Floras at the poles were cool to warm temperate, where large leaf-size was an adaptation to low light levels in the winter at high latitudes.
The early Cenozoic saw the evolution of grasses, but it was not until \(10-20\ {\rm Ma}\) that widespread grass-dominated ecosystems existed, even though they had started to form by a significant part of the global vegetation by \({\sim}25\ {\rm Ma}\). The spreading of grasslands was promoted in part by the cooling and increasing aridity. Many grasses have drought resistant adaptations (e.g., increased root growth, decreased shoot growth, reduced physiological activity during droughts, etc.), which coped with stress arising from the increased frequency of fires associated with a more arid climate.
6.3.2.8. Terrestrial Faunae#
Amphibians dominated the Carboniferous coal forests and continued as important aquatic animals during the Permian. During the Carboniferous (\({\sim}325\ {\rm Ma}\)), the first truly terrestrial vertebrates (the reptiles) evolved, where several major lineages radiated in the Late Carboniferous, but a relatively low diversity and small size. Two lineages emerged: the diapsids (the group containing living reptiles, dinosaurs, and birds) and the synapsids (mammal-like reptiles), where the latter were the dominant terrestrial vertebrates during the late Paleozoic.
Fossil remains indicate the synapsids had spread across Pangea by the Permian. The first mammal-like reptiles that evolved in the Late Carboniferous were the pelycosaurs. Unlike the earliest reptiles, pelycosaurs possessed a much larger head and body. Among them were the first terrestrial vertebrate carnivores. The edaphosaurs were herbivorous and sported huge, fin-like sails on their backs. Extremely long neural spines projecting from their backbones supported a then membrane. A rich supply of blood vessels at the base of the spines suggest that the sail functioned as a thermoregulator, allowing rapid absorption or radiation of heat. By the late Permian (\({\sim}260\ {\rm Ma}\)), pelycosaur diversity had declined and they were replaced with a new group of mammal-like reptiles, the therapsids. These animals looked like a cross between a hippopotamus and a crocodile. Unlike pelycosaurs, therapsids had limbs set beneath their bodies, giving them greater mobility and therefore increasing their foraging ranges.
During the Mesozoic, there was an evolutionary revolution in terrestrial vertebrates that rivaled the one occurring in the marine realm. Many new groups evolved and radiated during the Triassic: turtles, crocodilians, dinosaurs, and mammals later. Moreover, the first flying vertebrates (the pterosaurs) also evolved during the Late Jurassic (probably from theropod dinosaurs) and diversified greatly during the Cretaceous. During the early Mesozoic, many families of terrestrial tetrapods were global in their distribution, despite the break-up of Pangea.
More specific changes are evident in one of the dominant reptilian groups, the dinosaurs. Ornithopods (bipedal herbivores) evolved near the Late Triassic-Early Jurassic boundary (\({\sim}200\ {\rm Ma}\)). Diversity increase was limited until the Late Cretaceous, which was particularly evident in the hadrosaurs. This is attributed to the evolution of more efficient jaw mechanics and development of cutting teeth. This particular trend was probably in response to environmental stimuli, especially the changing nature of the plant food.
The Late Cretaceous diversification of angiosperms also corresponds strongly with insect diversification. Early Cretaceous flower have features such as stamens with small anthers and pollen grains too big for effective wind dispersal, which indicates that many were pollinated by insects. Moths and butterflies also seem to have radiated about the time of the angiosperms, as well as bees, whose fossil record dates back to the mid-Cretaceous.
Like reptiles that diversified in both marine and aerial niches during the Mesozoic, mammals similarly diversified during the Cenozoic, with the evolution of both cetaceans (whales) and bats. Terrestrial faunae are particularly characterized by a great increase in diversity of mammals (in terms of both morphology and size). Like the flora, the evolution of the terrestrial mammal fauna was strongly influenced by profound climatic changes of the Cenozoic. Many mammalian lineages increased in body size, in part in response to ecological changes brought on by the transition from a warm greenhouse world to an increasingly ice house world.
The classic example for this is the evolution of horses, from the small tropical-forest inhabiting forms to larger forms adapted for wide open grass plains and with teeth modified to eat this coarser vegetation. Many of the changes in diversity also relate to the shifting continents, and the evolution of increasingly more endemic groups, such as the many marsupial families that evolved on the Australian continent once it had separated from Antarctica.
Mammals (in general) have large brains for their body size, compared with other vertebrates (with the exception of birds). The evolution of the largest relative brain size occurred in the hominin genus Homo. Because the brain is a metabolically “hungry” organ, for hominids to evolve large brain-size, developmental tradeoffs were necessary to allocate energy that would enable larger brain growth. This is thought to have occurred by a reduction in gut size, which necessitated a change in diet, predominantly from herbivorous to omnivorous, with a large intake of meat. Increase in brain size in the Homo lineage correlates with a reduction not only in gut size, but also in jaw and tooth size.
The final species in this lineage, Homo sapiens, evolved such a well-developed neocortex and increased so much in cognitive development that it has not only manipulated the environment to a greater extent than any other single species of metazoan. The overall trend of evolution:
colonization first of the oceans,
then the terrestrial environment, and
then the atmosphere,
has been taken one step further by this species with the ability to spread beyond the atmosphere into outer space.
The evolution of life on planet Earth has not been a series of continuously random episodes that have fortuitously produced the pattern we see in the fossil record. For the last \(3.5\ \rm Gyr\) or so, life has expanded along environmental gradients (e.g., deep to shallow water; aquatic to terrestrial; terrestrial to aerial). The genetic changes that have occurred have been heavily constrained along pre-existing developmental pathways, resulting in many examples of convergent evolution. If we are to seek life on other planets, then we need to find another active, evolving planet.
How inevitable is life on a planet that has evolved in the way that Earth has?
If we were to re-run the clock on Earth, would we get a similar evolutionary scenario?
Conway Morris (2003) has argued that we would, and that ultimately what we perceive as intelligent life would also be inevitable. If another planet is evolving under very different constraints, would life evolve and would it bear any remote resemblance to life on Earth? This is a question that is still hotly debated in the exoplanet era of astrobiology.
6.4. Homework#
Problem 1
What factors on the early Earth are important for the emergence of life? Explain how the environment affects these factors, especially with respect to the development of metabolism.
Problem 2
Compare and contrast the metabolic processes of anabolism and catabolism. Describe the types of organisms that utilize each metabolic process.
Problem 3
How is energy actually stored and transferred in biology? Identify the chemical reactions, Gibbs free energy yields, and requisite environments for the three major forms of respiration.
Problem 4
Summarize the reductive acetyl-CoA pathway and why it is important for the earliest metabolic processes.
Problem 5
Describe the process of photosynthesis qualitatively and chemically. Is water a necessary prerequisite for photosynthesis? Explain your answer.
Problem 6
What does the fossil record suggest for the origins of the earliest communities of life on the Earth? What kind of physiological structures were common among these communities?
Problem 7
Summarize what we know about the limits of Earth-like life and why do we use the Earth as our primary model in the search for live elsewhere.
Problem 8
Identify the extreme environments for which life can unexpectedly thrive in. Is the presence of water a necessary or sufficient for the existence of life?
Problem 9
What were the most common types of lifeforms in Earth’s history? Describe how we identify the remains of lifeforms that are without shells or skeletons.
Problem 10
Summarize how life diversified after the Cambrian “Explosion”. What morphological and physiological changes occurred during the fish-tetrapod transition?
Problem 11
Describe how changes to Earth’s surface over the last \(500\ \rm Myr\) have influenced the types of lifeforms that have flourished or perished in response to an evolving Earth.