Life and its Requirements
Contents
4. Life and its Requirements#
4.1. Does ‘Life’ Have a Definition?#
The question “What is life?” is foundational to biology, but it is especially important to astrobiologists searching for signs of life in the cosmos. Previous attempts to define life have faced serious counterexamples, and may generate as many problems as they solve. To answer the question “What is life?”, we require a general theory of the nature of living systems. In absence of such a theory, we like alchemists during the European Enlightenment trying to define water before the advent of atomic theory. The best description of water at the time would be to list water’s properties:
wet,
transparent,
odorless,
tasteless,
thirst quenching, and
a good solvent.
Although, finding water that was odorless and tasteless might have been a struggle for the average 16th century investigator. No amount of (macroscopic) observational or conceptual analysis of these features will reveal that water contains two hydrogens and one oxygen (\(\rm H_2O\)). The most scientifically informative answer to the question “What is water?” is most definitely \(\rm H_2O\). In the absence of a good theory of the nature of living systems, the best we can do is to describe its features until we have a more precise intuition.
4.1.1. Attempts to Define ‘Life’#
There have been many attempts to define life dating back at least to Aristotle, who defined life in terms of the capacity to reproduce. To this day, there is not a broadly accepted definition of life, where the scientific literature is filled with suggestions. Carl Sagan catalogued physiological, metabolic, biochemical, Darwinian (or genetic) and thermodynamic definitions, along with their counterexamples. All attempts typically face problems, in that they include phenomena that most are reluctant to consider alive, or exclude entities that clearly are alive. Figure 4.1 shows a definition, but it actually relies on many definitions to form a complete picture.
A metabolic definition could be based on the ability to consume and convert energy in order to move, grow, or reproduce. But fire might be said to satisfy some or all of these criteria.
A thermodynamic definition might describe a living system as one that takes in energy in order to create order locally, but this would include crystals that are not generally considered alive.
A biochemical definition would be based on the presence of certain types of biomolecules, yet one must worry that any such choice could in the future face exceptions when the category of favored biomolecules changes. In that case, once living things would become non-living.
Genetic or Darwinian definitions are systems that are capable of evolution by natural selection, but these also face drawbacks. The taming (i.e. altered behavior) of wolves into dogs appears to fit this definition, but a behavior is not considered alive by itself.

Fig. 4.1 Definition of cellular life according to Budisa, Kubyshkin, & Schmidt (2020).#
The philosophical question of the definition of life has increasing practical importance, as laboratory experiments approach the synthesis of life, and as greater attention is focused on the search for life within the Solar System and beyond. Definitions of life are often explicit or implicit in planning remote in situ searches for extraterrestrial life. The design of life-detection experiment that could be performed on Europa or Mars by spacecraft landers depends on decisions about what life is, and what observations will count as evidence of a detection.
4.1.1.1. Viking’s Search for Life on Mars#
The Viking mission’s search for life on Mars in the mid-1970s was the first dedicated in situ search for extraterrestrial life. The basic approach was to conduct experiments with the martian soil to test for the presence of metabolizing organisms. The results of the labeled release experiment (see the LR in Fig. 4.2) in particular were not unlike what had been expected for the presence of life. In the end, the Viking biology team’s consensus was for a nonbiological interpretation, which was strongly influenced by the failure of the Viking gas chromatograph mass spectrometer (GCMS) to find any organic molecules to its limits of detection in the soil with sample heating up to \(500\ {\rm ^\circ C}\).
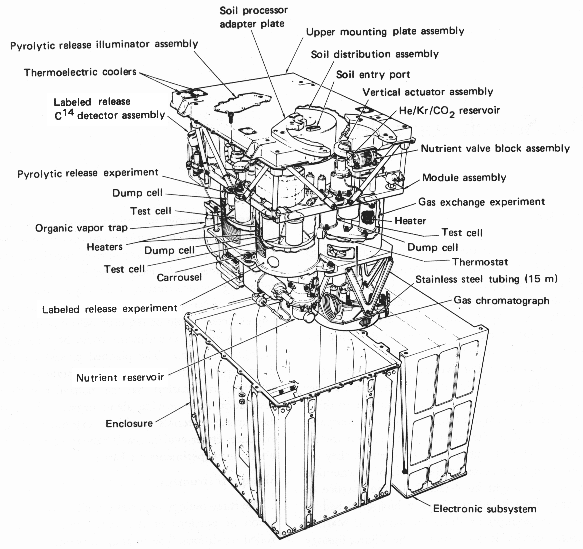
Fig. 4.2 NASA Viking Lander Biological Experiment Package. Image Credit: Wikipedia:Viking lander biological experiments/NASA.#
The GCMS was not intended to conduct a “life-detection” experiment, but actually did so by implicitly employing a biochemical definition. The GCMS would not have detected as many as \({\sim}10^6\) bacterial cells per gram of soil. It now appears that oxidation of the meteoritic organics on the martian surface may have produced nonvolatile organic compounds that would not have been easily detectable. The result was psychologically powerful: no (detected) organics, no life. A lesson learned is that any in situ search for extraterrestrial life should employ more than one definition of life so that results can be intercompared. If there were really one correct known definition of life, then the extra effort and design would be unnecessary.
4.1.1.2. The Darwinian Definition#
Darwinian (sometimes called genetic) definitions of life hold that life is a system capable of evolution by natural selection. One popular version is the chemical Darwinian definition, where life is a self-sustained chemical system capable of Darwinian evolution. The notion of Darwinian evolution includes processes of self-reproduction, material continuation over a historical lineage (i.e., gene transfer), genetic variation, and natural selection. The requirement the system is self-sustained refers to living systems that contain all the genetic information necessary for their own constant production (i.e., metabolism). The chemical Darwinian definition excludes computer or artificial life through its demand that chemical exchanges are responsible for the self-sustaining property. It also excludes biological viruses by virtue of the self-sustained requirement.
Some researchers (e.g., Dawkins (1983); Dennett (1995)) do not restrict Darwinian evolution to chemical systems, explicitly leaving open the possibility of computer life. This characterizes Darwinian evolution by how it functions, or how it is more a general process that can be abstracted from any particular physical realization. In this functionalist view, it is not the computer that is alive but rather the processes themselves. This is similar to how energy propagates through a medium using waves, where the material at the wave source does not interact directly with the receiver. The artificial vehicle of the computer has a status no different from that of the artificial glassware that might be used in a laboratory synthesis of organic life. A computer simulation of cellular biochemistry is a simulation of biochemistry, and not biochemistry itself. No computer simulation of photosynthesis is actually photosynthesis since it does not yield authentic carbohydrates. In the functionalist view, the simulation is life because life is an abstract process independent of any particular physical realization.
There are other problems with Darwinian definitions, where it is possible (though not generally favored) that early cellular life on Earth or some other work passed through a period reproduction without DNA-type replication and Darwinian evolution could not yet operate (e.g., Dyson (1985)). In this hypothesis, protein-based creatures capable of metabolism predated the development of exact replication based on nucleic acids. If such entities, where discovered on another world, then Darwinian definitions would preclude them from being considered alive. For more recent details (as of 2016), see How to build life in a pre-Darwinian world.
A simple objection to the Darwinian definition considers that individual sexually reproducing organisms in our DNA-protein do not themselves evolve and would categorize many living entities in our world are not examples of life. The Darwinian definition refers to a system that at least in some cases must contain more than one entity, which would preclude Victor Frankenstein’s unique creation from being considered alive, even though the tale clearly describes a living entity. A practical drawback to Darwinian definitions is that they depend on how long a system should wait before demonstrating that it is capable of Darwinian evolution. How do we decide what are the proper conditions for a system to be capable of Darwinian evolution?
4.1.2. Definitions#
Definitions are concerned with language and concepts, at least philosophically. For example, the definition of a “bachelor” is an unmarried human male, which explains the meaning of the word by dissecting the concept that we associate with it. Every definition has two parts: (1) the definiendum is the expression being defined and (2) the definiens is the expression doing the defining.
4.1.2.1. Varieties of Definition#
Lexical definitions report on the standard meaning of terms in a natural language (e.g., dictionary definitions). Lexical definitions are in contrast to stipulative definitions, which explicitly introduce new meanings for terms. The stipulative definition from physics for the term ‘work’ refers to the magnitude of an acting force and the displacement due to its action. Stipulative definitions are also used to introduce invented terms (e.g., electron or gene). Unlike lexical definitions, stipulative definitions are arbitrary in the sense that rather than reporting on the existing meanings of terms, they explicitly introduce new meanings.
Another type of definition is the ostensive definition that specify the meaning of a term merely by indicating a few prototypical examples within its extension, or the class of all things to which it applies. An adult who explains the meaning of the word ‘dog’ to a child by pointing to a dog and saying “that is a dog” is providing an ostensive definition.
Operational definitions are like ostensive definitions, where they explain meanings through representative examples. Operational definitions do not directly indicate examples, but instead specify procedures that can be performed on something to determine whether it falls into an extension of the definiendum. For example, an acid can be operationally defined as something that turns litmus paper red. The definiens specifies a procedure that can be used to determine whether an unknown substance is an acid. Some astrobiologists (e.g., Chyba & McDonald (1996); Conrad & Nealson (2001)) have called for the use of operational definitions in searches for extraterrestrial life. The problem with operational definitions is that they do not tell one very much about what the items falling under the definiendum have in common. The fact that litmus paper turns red when placed in a liquid doesn’t tell us much about the nature of acidity, only that a particular liquid is something called acid.
The most informative definitions specify the meanings of terms by analyzing concepts and supplying a noncircular synonym for the term begin defined. In philosophy, such definitions are know as full or complete definitions. Philosophers sometimes uses these expressions to designate more fine-grained distinctions, in which the term ideal definition is used instead.
4.1.2.2. Ideal Definitions#
Ideal definitions explain the meanings of terms by relating them to expression that we already understand. It is important that the definiens make use of neither the term being defined nor one of its close cognates; otherwise the definition will be circular (e.g., defining a line as a linear path or a cause as something that produces an effect). Someone that does not understand the meaning of the definiens ‘cause’ will also not understand the meaning of ‘effect’ since the definition of ‘effect’ may be something that is caused.
The definition of ‘bachelor’ is not circular since the concept of being unmarried, human, and male does not presuppose an understanding of the concept of bachelor. The definiens provides an informative analysis of the meaning of the definiendum ‘bachelor’. An ideal definition specifies the meaning of a term by reference to a logical conjunction of properties as opposed to representative examples (i.e., ostensive definition), or a procedure for recognizing examples (i.e., operational definition). The conjunction of descriptions determines the extension of the definiendum by specifying necessary and sufficient conditions for its application.
A necessary condition for falling into the extension of a term is a condition in whose absence the term does not apply. For example, “Without a microscope, a person cannot see viruses.”
A sufficient condition is a condition in whose presence the term cannot fail to apply. For example, “For a shape to be a square, each of its sides is equal in length to each of the other sides.”
Most proposed ideal definitions face borderline cases in which it is uncertain as to whether something satisfies the conjunction of predicates supplied by the definiens. A good example is the question of whether a ten-year-old boy is a bachelor. Even if this case is resolved by adding an additional condition (e.g., adult) to the definiens, there wil be other borderline cases because language is vague.
Try to distinguish a bald man from a man who is not bald in terms of the number of hairs on his head. the fact that we cannot specify a crisp boundary does not show that there is no difference between being bald and not being bald. Ideal definitions that specify necessary and sufficient conditions are rare. We can often construct fairly satisfactory approximations of an ideal definition despite the problem of borderline cases. If definitions of life faced nothing more serious than counterexamples (e.g., viruses), there might not be insurmountable problems. Good definitions of life must deal with quartz crystals and candle flames, which are presumably not alive.
4.1.3. Natural Kinds#
Ideal definitions specify meaning by providing a complete analysis of the concepts associated with terms. However, they do not supply good answers to question about the identity of natural kinds, or categories carved out by nature. It is important to astrobiology because it seems likely that life is a natural kind term and represents an objective fact about the natural world.
Consider trying to answer the question “What is water?” by defining the natural kind term ‘water.’ The term ‘water’ could be defined by reference to its sensible properties (see Sect. 4.1). This definition of ‘water’ is not simply a matter of linguistic convention. A reference to a list of sensible properties cannot exclude things that superficially resemble water but are not in fact water. For example, the alchemists identified nitric acid (known as aqua fortis, or strong water) and mixtures of hydrochloric acid (known as aqua regia, or royal water) as water. A mixture of alcohols was called aqua vitae, or water of life. Even today, we classify various liquids in terms of their sensible properties (e.g., salt water, muddy water, and distilled water). Which of the sensible properties (e.g., transparency or tastelessness) are more important to the definition of water?
And so it [water] is sometimes sharp and sometimes strong, sometimes acid and sometimes bitter, sometimes sweet and sometimes thick or thin, sometimes it is seen bringing hurt or pestilence, sometimes health-giving, sometimes poisonous. So one would say that it suffers change into as many natures as are the different places through which it passes. And as the mirror changes with the colour of its object so it changes with the nature of the place through which it passes: health-giving, noisome, laxative, astringent, sulphurous, salt, incarnadined, mournful, raging, angry, red, yellow, green, black, blue, greasy, fat, thin.
—Leonardo da Vinci (1513)
Without an understanding of the intrinsic nature of water, there is not a definitive answer to the question “What is water?” But an understanding of the molecular structure of matter causes such quandaries to disappear. The identification of water with \(\rm H_2O\) explains why liquids can resemble water and not be water because their molecular composition is more than \(\rm H_2O\) alone. In addition, the identification holds regardless of whether the water is in any of its familiar forms (solid, liquid, or gas), and it wil hold equally well in the less familiar high-pressure phases. The Aristotelian view of water as one of the basic elements began to fall into disfavor with a paper from Antoine Lavoisier (1783) that showed water is not an element, but can be decomposed and recombined.
The identification of water with \(\rm H_2O\) does not have the character of an ideal definition. It cannot be viewed as explicating the concept that has historically been associated with the term ‘water’ because that concept encompasses stuff varying widely in chemical and physical composition. The claim that water is \(\rm H_2O\) began as a testable empirical conjecture, and it is now considered so well confirmed that most scientists characterize it as a fact.
4.1.4. What is Life?#
If life is a natural kind, then attempts to define life are fundamentally misguided. Definitions serve only to explain the concepts that we currently associate with terms. Concepts cannot reveal the objecting underlying nature (or lack thereof) of the categories designated by natural kind terms. It is the underlying nature (not the concepts in our heads) that we are interested in when we use a natural kind term. The term ‘water’ means whatever the stuff in streams, lakes, oceans, and everything else that is water has in common. We currently believe that this stuff is \(\rm H_2O\), and our belief is based on a well-confirmed, general scientific theory of matter.
We cannot be absolutely positive that molecular theory is the final word on the nature of matter; conclusive proof is just not possible in science. Our current scientific concept of water as \(\rm H_2O\) represents a vast improvement over earlier concepts based on superficial sensory experience. If we someday discover that molecular theory is wrong, we will change the concept that we associate with ‘water,’ but we will still be talking about the same thing.
We could define ‘life’ to mean whatever cyanobacteria, hyperthermophilic archaeobacteria, amoeba, mushrooms, palm trees, sea turtles, elephants, humans, and everything else that is alive (on Earth or elsewhere) has in common. No purported definition of ‘life’ can provide a scientifically satisfying answer to the question “What is life?” because no mere analysis using human concepts can reveal the nature of a world that lies beyond them. The best we can do is to construct and empirically test theories about the general nature of living systems. We want theories that settle our dilemmas in classification by explaining puzzle cases:
Why things that are alive sometimes lack features that we associate with life?
Why things that are non-living sometimes have features that we associate with life?
No scientific theory can be conclusive, but someday we may have a well-confirmed, adequately general theory of life that will allow us to formulate a theoretical identity statement (e.g., “water is \(\rm H_2O\)”) providing a scientifically satisfying answer to the question “What is life?”
4.1.4.1. Dreams of a General Theory of Life#
To formulate a convincing theoretical identity statement for life, we need a general theory of living systems. Our current sample size for such a theory is one (i.e., terrestrial life), which is a problem. Although the morphological diversity of terrestrial life is extraordinary, all known life on Earth is also extraordinarily similar in its biochemistry. With the exception of some viruses, the hereditary material of all known life on Earth is DNA of the same right-handed chirality. Life on Earth utilizes 20 amino acids to construct proteins, and these amino acids are typically of left-handed chirality. These biochemical similarities lead to the conclusion that life on Earth had a single origin.
Darwinian evolution then explains how this common biochemical framework yielded such an amazing diversity of life. It is difficult to decide which features of terrestrial life are common to all life (wherever it may be found) because the biochemical similarities of all life on Earth can be explained in terms of a single origin. Many biochemical features that strike us as important may derive from mere chemical or physical contingencies present at the time life originated on Earth (Sagan (1974)).
How can we discriminate the contingent from the essential? It is a bit like trying to come up with a theory of mammals when one can observe only zebras. What features of zebras should one focus upon (e.g., their stripes or mammary glands)? The mammary glands (although present in only some zebras) tells us more about what it means to be a mammal than do the ubiquitous stripes. Without access to living things with a different historical origin, it is difficult to formulate an adequately general theory of living systems. This problem is not unique to life, where it reflects a simple logical point. One cannot generalize from a single example. Biochemical analyses coupled with knowledge of evolution suggests that much of the diversity observed on Earth may be a historical accident. Had the history of the Earth been different, life on Earth would certainly be different. In essence, the common origin of contemporary terrestrial life blinds us to the possibilities for life in general.
Many definitions of life cite sensible properties of terrestrial life, such as metabolism, reproduction, complex hierarchical structure, and self-regulation. But defining life in terms of sensible properties is analogous to defining water as being wet, transparent, tasteless, etc. Reference to sensible properties for water is unable to exclude things that are not water and to include everything that is water (e.g., ice).
4.1.4.2. How to Search for Extraterrestrial Life#
There remains the problem of how to hunt for extraterrestrial life without either a definition of life or a general theory of living systems. One approach is to treat the features that we currently use as tentative criteria for life. The features will then necessarily be inconclusive, where their absence cannot be taken as sufficient for concluding that something is not alive. Therefore, they cannot be viewed as providing operational definitions of life. The purpose of using tentative criteria is not to definitively settle the issue of whether something is alive, but rather to focus attention on possible candidates (i.e., physical systems whose status as living or non-living is genuinely unclear).
The criteria should include a wide diversity of features of terrestrial life, where diversity is absolutely crucial when one is looking for extinct extraterrestrial life (e.g., in the martian meteorite ALH84001, or with instrument packages delivered to ancient martian flood plains). Some feature for shaping searches for extraterrestrial life may not even be universal to terrestrial life. For example, features that are common only to life found in certain terrestrial environments may prove more useful for searching for life in analogous extraterrestrial environments than are features that are universal to terrestrial life. Features that are common or non-existent among non-living terrestrial systems may make good criteria for present or past life because the stand out against the background of non-living processes. The chains of chemically pure, single-domain magnetite crystals found in ALH84001 provide a potential example. If it turns out that these chains can only be produced biogenically, then they will provide a good biosignature for life, despite the fact that most terrestrial bacteria do not produce them.
The basic idea behind our strategy for searching for extraterrestrial life is to employ empirically well-founded (albeit provisional) criteria that increase the probability of recognizing extraterrestrial life while minimizing the chances of being misled by inadequate definitions. This is similar in spirit to suggestions that in situ searches for extraterrestrial life should rely when possible on contrasting definitions of life. The important point is to include the potential boundaries of our current concept of life. It is only in this way that we can move beyond our geocentric ideas and recognize genuinely weird extraterrestrial life, should we be fortunate enough to encounter it.
4.1.5. Requirements and Limits for Life in the Context of Exoplanets (McKay 2014)#
4.1.5.1. Limits to Life#
There are two somewhat different approaches to the question of the limits to life:
to determine the requirements for life
to determine the extreme environments in which adapted organisms (i.e., extremophiles) can survive.
The requirements for life on Earth broadly include four items: (1) energy, (2) carbon, (3) liquid water, and (4) various other elements. In our Solar System, it is the occurrence of liquid water that appears to limit the occurrence of habitable environments, and it appears to be the case for exoplanetary systems as well.
The first requirement arises from basic thermodynamic considerations, where it is clear that life requires a source of energy. Life on Earth uses only one energy source: that associated with the transfer of electrons by chemical reactions of reduction and oxidation to power metabolism and growth. Methane-producing microbes use the reaction of \(\rm CO_2\) with \(\rm H_2\) to produce \(\rm CH_4\). Photosynthetic organisms use a light-absorbing protein (e.g., chlorophyll, bacteriochlorophylls, and bacteriorhodopsin) to convert photon energy to the energy of an electron which then completes a redox reaction. This occurs in the mitochondria in of most eukaryotes and in the cell membrane of prokaryotic cells. Although life can detect and generate other energy sources (including magnetic, kinetic, gravitational, thermal gradient, and electrostatic), none of these is used for metabolic energy.
Carbon has the dominant role as the backbone molecule for Earth life and is widespread in the Solar System. However, carbon may not be that useful as an indicator because the Earth is significantly depleted in carbon compared with the outer Solar System. The vast majority of the carbon on Earth is stored in sedimentary rocks within the crust. However, light carbon-containing molecules (e.g., \(\rm CO_2\), \(\rm CO\), and \(\rm CH_4\)) are volatile, which means adequate carbon is present at the surface of the terrestrial planets.
Life on Earth uses a vast array of the elements available at the surface. However this does not prove that these elements are absolute requirements for carbon-based life in general. The elements \(\rm N\), \(\rm S\), and \(\rm P\) are probably the leading candidates for the status of required elements. If liquid water and biologically available nitrogen are present, then phosphorous, potassium, sodium, sulfur, and calcium might come next, as these are the most abundant elements in bacteria. However, there is no definitive list and habitability can only be confirmed by showing inhabitation.
4.1.5.2. Strategy for Exoplanets#
The second approach to the requirement for life is based on the abilities of extremophiles in a range of environmental factors (see Table 4.1) It may seem logical to focus on primary production because without that there cannot be an ecosystem. However, its is possible that photochemical processes in an exoplanet atmosphere play the roe of primary production as has been suggested for Titan. The most important parameter for Earth-like life is the presence of liquid water, which directly depends on pressure and temperature. Temperature is key because of its influence on liquid water and it can be directly estimated from orbital and climate models of exoplanetary systems.
Parameter |
Limit |
Note |
---|---|---|
Lower temperature |
\({\sim}-15\ {\rm ^\circ C}\) |
Limited by liquid water associated with thin films or saline solutions |
Upper temperature |
\(122\ {\rm ^\circ C}\) |
Solubility of lipids in water, protein stability |
Maximum pressure |
\(1100\ {\rm atm}\) |
|
Low light |
\({\sim}5 \times 10^{-6}\) direct solar flux on Earth |
Algae under ice and deep sea |
pH |
\(0-12.5\) |
|
Salinity |
Saturated \(\rm NaCl\) |
Depends on the salt and temperature |
Water activity |
0.6 (0.8) |
Yeast and molds (bacteria) |
UV Radiation |
\(\geq 1000\ {\rm J/m^2}\) or \(50\ {\rm Gray/hour}\) |
D. radiodurans growth with continuous dose |
Temperature, Cold limit: Many organisms can grow and reproduce at temperatures well below the freezing point of pure water because their intracellular material contains salts and other solutes that lower the freezing point of the solution. The snow algae Chlamydomanas nivalis thrives in liquid water associated with snow (coloring it red), but the algae are the beneficiaries of external processes that melt the snow.
Temperature, Hot limit: As water is heated (and maintained as a liquid under pressure), the dielectric constant and the polarity of the liquid decreases sharply. This significantly changes the characteristics of water as a solvent and it interaction with dissolved biomolecules (in particular lipids, but also proteins and nucleic acids). Organisms that can survive the highest temperatures are archaea, as their membrane lipids are held together with ether bonds.
Water, Dry limit: On worlds where the temperature is within the appropriate range (for Earth-like life), life may be limited by the availability of water (i.e., low water activity), where we only have to look at Mars for an example. In dry environments on Earth, photosynthetic cyanobacteria and lichens survive in several dry deserts. Endolithic cyanobacteria live just below the surface of halite rock in the dry core of the Atacama Desert.
Energy: Energy for life can come from chemical redox couples generated by geothermal processes or light from the central star. Geothermal flux can arise from (i) the planet cooling off from its gravitational heat of formation, (ii) decay of long-lived radioactive elements, or (iii) tidal heating for a close-orbiting world or moon. On Earth only a tiny fraction of the geothermal heat is converted into chemical energy, whereas about half the solar flux occurs at wavelengths that are usable for photosynthesis. Life is able to use light at very low levels, as red macroalgae on deep seamounts grows at low light levels.
UV and Radiation: Complex lifeforms (e.g., humans) are sensitive to radiation but the does that can be tolerated by many microorganisms is astonishingly high. A well-studied soil heterotroph with high radiation tolerance is Deinococcus radiodurans, which can withstand an acute dose of radiation due to adaptation to dehydration stress.
4.2. Evolution: a Defining Feature of Life#
4.2.1. From Lamarck to Darwin to the Central Dogma#
The basic notion of evolution is that inherited changes in populations of organisms result in expressed differences over time. These differences are at the gene level (i.e., genotype) and/or expression of the gene into an identifiable characteristic (i.e., phenotype). The important underlying fact of evolution is that all organism share a common ancestor. We see this in the universal nature of the genetic code and in the unity of biochemistry:
all organisms share the same biochemical tools to translate the universal information code from genes to proteins,
all proteins are composed of the same twenty essential amino acids, and
all organisms derive energy from metabolic, catalytic, and biosynthetic processes from the same high energy organic compounds (e.g., adenosine triphosphate (ATP)).
Darwin’s On the Origin of Species describes his theory of evolution using evidence that included an ancient Earth, which geologists at the time (in 1859) believed to be in the millions of years. Darwin also took the extinction of species to ba a real phenomenon since fossils existed that were without living representatives. Since different species showed close phenotypic similarities, he argued that existing organisms descended from other organisms including extinct groups.
The key to his evolutionary theory was that inherited characteristics of organisms can change through time and that these changes occurred gradually and without discontinuities. Jean-Baptiste Lamarck recognized (in 1809) a similar principle of evolution and offered an explanation generally referred to as inheritance of acquired characters. Lamarck meant that the variation in characteristics or adaptations seen in organisms were acquired in response to the environment. Classic examples include the long neck of the giraffe as an adaptation for foraging tender foliage on treetops, or the use of long legs by some aquatic fowl to venture into deep waters in search of prey. Under Darwinian principles they were not acquired as a response to the needs of the environments.
One of Darwin’s major contributions was his explanation of how and why organisms change over time and how they acquire characteristics useful for living in different environments. Darwin referred to the mechanism for character changes through time as natural selection. Natural selection is based on the idea of the struggle for existence (i.e., survival of the fittest) in populations where there are more individuals of each species than can survive. A variation in any characteristic of an individual that gives an advantage in surviving (and reproducing) will be “naturally selected” since the new trait will be preferentially inherited by subsequent generations (see Fig. 4.3 or Khan Academy for more detail). Darwin differed from Lamarck by recognizing that character changes that offer a survival advantage and are “naturally selected” originate from a pool of randomly generated character changes that are not directed by environmental conditions.
Note
Darwin proposed his theory without knowing the mechanism for the inheritance of acquired traits. Forty years later, the field of genetics would begin with the recognition that Gregor Mendel’s principle of discrete units of inheritance (i.e., genes) was correct.

Fig. 4.3 Diagram of mutation and selection in evolution. Image Credit: Wikimedia Commons:mutation and selection diagram.#
Mendelian genetics established that phenotypes are transmitted from one generation to another following statistical principles and that these phenotypes reside in simple heritable “characters.” The nature of these heritable characters were unknown to Mendel but their location was confined to chromosomes by 1910 and then to DNA as the genetic material by Hershey & Chase (1952). This immediately led to the discovery by Watson & Crick (1953) of the double-helix structure of DNA. Shortly afterwards the concept of the genetic code was developed with the understanding that a gene is primarily a sequence along a section of DNA that codes for a protein using an alphabet composed of the four bases that constitute DNA.
The steps leading from DNA to a specific protein are referred to as the central dogma: a DNA gene is transcribed to make messenger RNA (mRNA), followed by translation of mRNA into a protein. The exceptions to the central dogma are those genes that specify not proteins, but instead the various classes of RNA that are involved in both transcription and translation (e.g., ribosomal RNA (rRna) and transfer RNA (tRNA)).
4.2.2. Evolution at the Molecular Level#
The discovery by Watson and Crick opened the doors to studies of evolution at the molecular level and helped develop classification schemes that allow for the evolutionary comparisons of groups of extant organisms. Usually ribosomal RNA genes and ribosomal protein genes are used for evolutionary studies because they have highly conserved sequences (i.e., sequences that are found across all domains of life). Most functional RNA molecules have secondary structure that are associated with their function. Mutations that change the secondary structure of RNA molecules will frequently render them inactive. These conserved sequences must have originated a long time ago in a common ancestor and are fundamentally important to all species.
A central concept in evolutionary theory is that a gene coding for a characteristic is subject to mutation (i.e., change) in a random fashion, which in some cases can lead to variability in that characteristic in the next generation.
Mutations come about due to mistakes made during DNA replication, or through external factors such as ionizing radiation or toxic chemicals.
Most mutations have little or no effect on the protein product of the gene or the function of the RNA.
Those that involve deletions or insertions can result in structural changes in the transcribed protein or render structures inactive int the ribosomal RNA.
The most lethal mutations are those that damage the genes involved in DNA replication, transcription of DNA into mRNA, or translation of mRNA into a protein.
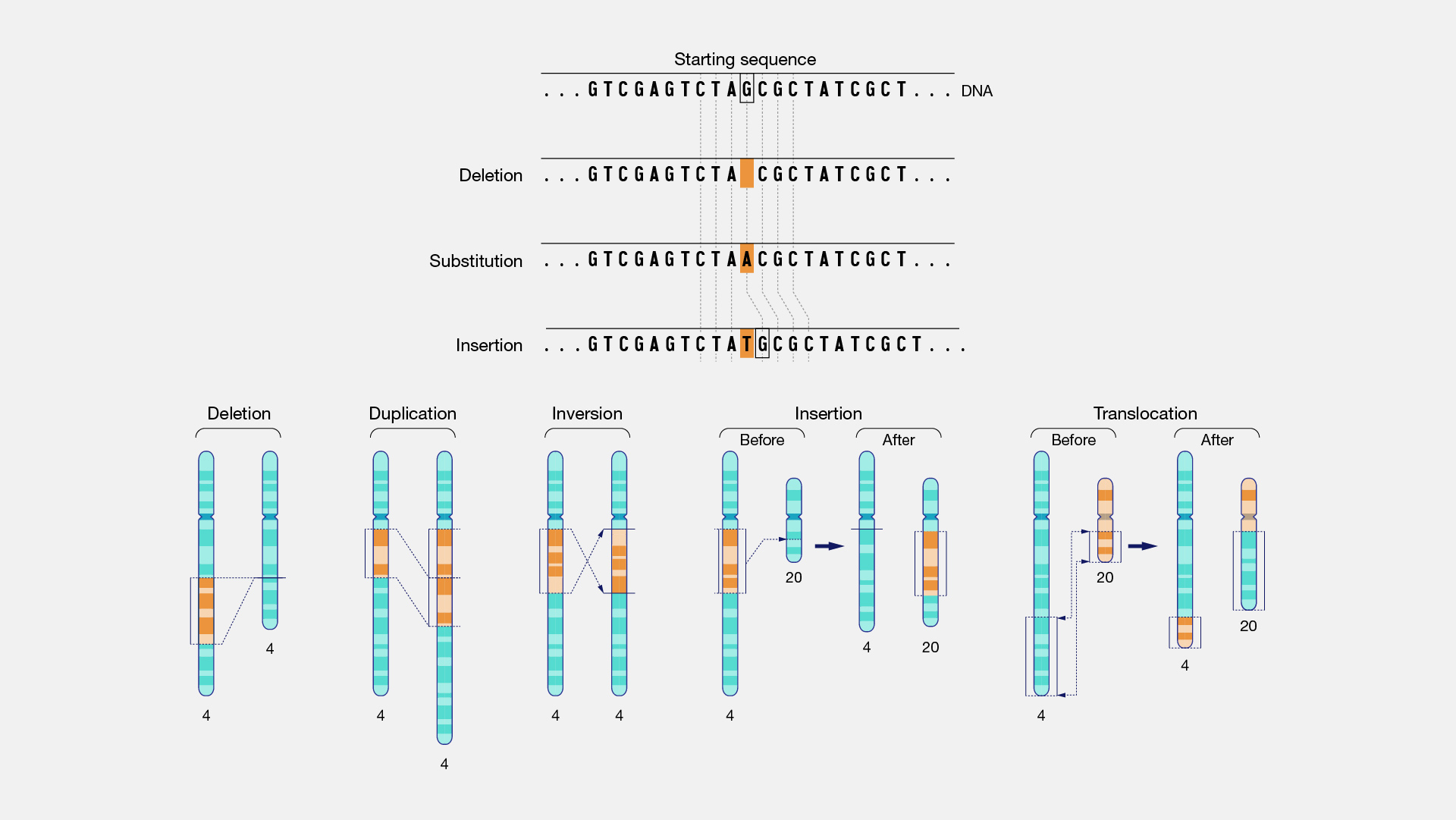
Fig. 4.4 Examples of mutation within the genome. Image Credit: National Human Genome Research Institute.#
Changing environmental conditions can negatively affect growth and survival (e.g., inducing stresses), which can result in the death of an organism depending on the degree and kind of stress. To survive a lethal stress, the organism must have mutation rates sufficiently high to handle the stress, but not so high as to cause lethal damage to the genome (i.e., the entire set of genes defining the species).
All extant organisms have a set of conserved genes for repairing mutations or proteins affected by environmental stresses (e.g., starvation, heat, radiation, changes in pH, etc.). Stress genes greatly reduce the number of deleterious mutations, while they are not 100% effective. The same genes can also targe other specific genes for an increased mutation rate under stress conditions, which are called stress-directed adaptive mutations. This mechanism can be observed when bacteria are starving, but undergo increased evolutionary rates of specific genes involved in the metabolism of alternative nutrients for growth.
In evolution, adaptation means more than simply being well suited to the environment, where it also involves (in any generation) the selection of one particular genetic change (over many other possibilities) that results in maximum reproductive success. Many incremental steps are involved in evolving complex structures and process, where it would seem that adaptation involves a sequence of coordinated (not random) steps. Until recently, there was no satisfactory mechanism that could account for the evolution of complex structures.
To kinds of evolutionary change are recognized.
Microevolution results in changes at the species level and accounts for the short term variability observed in populations.
Macroevolution involves the more substantial changes that over long time result in the development of a new hierarchy, or higher taxa (e.g., genera, families, orders, etc.). It affects the genotypes of individuals within populations and also involves microevolution. It is also invoked as the mechanism that results in the gradual formation of novel complex structures that involve multiple genes.
Development of the eye has provided a classic illustration for gradualism producing increasing complexity and function. There are more than forty different eye structures found in both invertebrates and vertebrates with a range of complexity from light sensitive patches to compound eyes. It was previously believed that these photosensitive organs developed independently along several different branches of the Tree of Life, and a classic example of convergent evolution (i.e., independent evolution of morphologically an/or functionally similar structures). In many cases macroevolution is not totally a gradual set of changes based on mutation and natural selection. There appears to be an common set of genes that instigated the evolution of the eye in a fruit fly, squid, and humans. These genes are called “tool box” genes, and are common to many diverse organisms, which implies that they are inherited from a common ancestor.
For example, one group of genes called HOX genes accounts for the incredibly high diversity found in animal body plans. The three principal anatomical plans for wings exemplified in birds, bats, and pterosaurs were also though to be products of convergent evolution.
The bird wing developed from the entire arm.
The bat wing developed from a hand.
The pterosaur wing from a single finger.
Similar HOX genes, acting on different sets of genes in birds, bats, and pterosaurs resulted in the evolution of different kinds of wings.
The profoundly important point is that the origin of diverse body forms of animals and their organs may have more to do with the way multiple genes are expressed and less to do with the number of different kinds of genes. We are learning that a basic set of genes is used in animals in different ways to produce the myriad different body forms, appendages, and organs.
The new combination of evolution with developmental biology is called Evo-Devo and is revolutionizing our understanding of macroevolution and embryology. Evo-Devo also offers an explanation for the rapid macroevolutionary changes (called punctuated equilibrium by Eldredge & Gould (1972)) that appear in the fossil record and that cannot be explained by gradualism. An example of punctuated equilibrium is the sudden appearance of diverse animal forms during the Cambrian explosion 550 million years ago (or 550 Ma). Evo-Devo studies indicate that this sudden emergence of highly diverse animal forms was due to the evolution of key regulatory HOX genes in the common ancestor to all Cambrian animals.

Fig. 4.5 The punctuated equilibrium model (top) consists of morphological stability followed by rare bursts of evolutionary change. In contrast, phyletic gradualism (below), is a more gradual, continuous model of evolution, with equilibrium states separated by jump phases. Image Credit: Wikipedia:punctuated equilibrium.#
Computer algorithms have been developed that are inspired by Darwinian evolution and are aptly named genetic algorithms due to how they can modify populations of data over generations. These algorithms mimic the process of natural selection by employing a fitness function, which evaluates data within the domain and modifies it based on a set of rules. The process of Darwinian evolution can be more efficient than a brute force method of sorting (see this blog for an example).
4.2.3. Mechanisms for Acquiring New Genes#
Other mechanisms (besides mutation) can effect changes in genes that coordinate cell structures, metabolism, or physiological traits through the sudden acquisition of new genes or incremental changes of individual genes or groups of genes. Theses mechanisms are:
fusion of different cells (i.e., endosymbiosis),
coevolution, and
lateral gene transfer.
Symbiosis is any interaction between two organisms in which at least one of the organisms benefits from the relationship. This broad definition includes parasitic associations in which the parasite benefits at the expense of the host, or mutualistic associations in which both organisms benefit. Some symbiotic associations are obligatory, where either the host or symbiont (or both) is unable to live independently.
For instance, the first eukaryote cell (i.e., cell with a nucleus) may have formed by the fusion of Bacteria and Archaea, where the Bacteria contributed the operational genes while the Archaea contributed the informational genes (Rivera & Lake (2004)). Such a fusion would fall into the category of mutualistic symbiosis since both cells benefited from this association. There is evidence that ancient symbioses in eukaryote cells, where their mitochondria (for oxygen respiration) and chloroplasts (for photosynthesis) occurred in specific groups of bacteria (see here for more details).
The proposed fusion of an archaeum with a bacterium somehow resulted in conditions favorable for evolution to greater complexity, multicellularity, and sexual reproduction. The later acquisition by early eukaryotes of the mitochondria and chloroplast from bacteria allowed eukaryotes to adapt into habitats with abundant light and oxygen (i.e., aerobic environment). Unfortunately, most of the evolutionary steps from the proposed fusion-based “proto-eukaryote” to single-cell eukaryotes are unknown since no known extant organism retains the intermediate characteristics.
The fusion of two cells is not believed to have been a common occurrence in the early life history of organisms, but there are many examples of other forms of symbiosis that are widely distributed in eukaryotes and allow them to live under conditions that would be otherwise impossible. One of the first cases was the symbiosis of an alga (singular of algae) and a fungus to form a lichen, which was recognized and researched in detail by Beatrix Potter (author of Peter Rabbit) well before symbiosis was accepted by the British scientific community.
Parasitism can result in radical changes in the physiology of the host that include mating and feeding behavior, as well as morphological changes. Coevolution is a special kind of symbiosis, in which two kinds of organisms interact in such a way that each exerts a selective pressure on the other. Classical examples include flowering plants and their insect pollinators, or predators with their prey. There may be traits in modern-day dogs that have coevolved due to their interactions with humans (Nagasawa et al. (2015)). Understanding the nature of coevolving ecosystems is one of the most difficult and important challenges in ecology.
Lateral gene transfer is the transfer of DNA from one organism to another such that the recipient is permanently changed in their genetic composition. Genetic exchange can be mediated by
cell-cell contact (i.e., conjugation),
viral infection (i.e., transduction), or
incorporation of DNA from the environment (i.e., transformation).
The recent accumulation of complete genome sequence from representatives of all the domains of life has revealed a universal pattern of lateral gene transfer for acquiring genes or parts of genes. We now know that viruses have played and continue to play a role in the evolution of life through lateral gene transfer (Canchaya et al. (2003)).
Note
A virus is defined as an intracellular parasite and is incapable of living without a host cell. While it shares many of the biochemical characteristics of a living cell including nucleic acids and proteins, it cannot reproduce independently, only by infecting a normal cell.
This is illustrated by the high abundance of bacterial viruses (i.e., bacteriophages or phages) in marine environments, exceeding the bacterial population by an order of magnitude. Viruses are the most abundant biological entity on Earth, yet are poorly understood. It is presumed that the primary role of viruses in the environment is causing death in bacteria (or producing disease in eukaryotes), but their significance as vehicle for transmitting new genes to bacteria in situ is not well understood, although likely extensive. Jiang & Paul (1998) calculated that at the low infection rate of \(10^{-8}\) per infected bacterial population, viral-mediated gene transfer takes place in Earth’s oceans at the rate of \({\sim}2\times 10^{16}\) per second.
How important is lateral gene transfer in evolution? Results from whole genome sequences of bacteria and archaea indicate that lateral gene transfer may be the most important mechanism for acquiring new genes, including those involved in complex and coordinated phenotypes. For example, \({\sim}16\%\) of the genome of Escherichia coli K12 is viral genes. Microorganisms have evolved elaborate mechanisms for incorporation of acquired genes into their chromosome at specific sites. These sites can serve as “pathogenicity islands” if all of the acquired genes are involved in disease production, or they may be “genetic islands,” which align acquired genes in involved in key physiological activities (e.g., magnetotaxis).
It is very unlikely that the formation of a genome with sufficient information to lead to free-living (self-sufficient) cells could have originated without a mechanism for acquiring “functional” genes from other early cells or communities of interdependent cells or “precells” (Baross & Hoffman (1985)). This is certainly consistent with the fact that all life on Earth is derived from a common ancestral pool of genes based on a universal genetic code. Darwinian evolution would have played an important role in these early stages and selection would have favored specific biochemical and molecular structures and mechanisms over others.
Could this imply that if we started over again by resetting the clock to 4 billion years ago (4 Ga), the resultant life would have the same biochemical and molecular properties (including the same genetic code) as present-day Earth life? If environmental conditions and the starting pool of organic compounds were the same, it is probable that a second genesis would result in biochemistry that would resemble or possibly be indistinguishable from present-day Earth life. In such a scenario, contingency in evolution could result in the selection of organisms and ecosystems significantly different from those found on Earth. Compared to present-day organisms, they would share a similar biochemistry and evolve many or all of the same phenotypes (both structural and functional) albeit possibly with different genotypes.
4.2.4. Could There be Life without Evolution?#
Many of the definitions of life include the phrase “undergoes Darwinian evolution.” The implication is that phenotypic changes and adaptation are necessary to:
exploit unstable environmental conditions,
function more optimally in the environment, and
provide a mechanism to increase biological complexity.
Evolutionary changes have even been suggested for hypothesized “clay crystal life” of Graham Cairns-Smith, referring to randomly occurring errors in crystal structure during crystal growth as analogous to mutations. Would a self-replicating chemical system capable of chemical transformations in the environment be considered life? If self-replicating chemical compounds are not life, then replication by itself is not sufficient as a defining characteristic of life. The ability to undergo Darwinian evolution is also not sufficient to define life if we consider minerals that are capable of reproducing errors in their crystal structure as equivalent to evolution. It is important to emphasize, that evolution is not simply reproducing mutations (mistakes in clays), but selecting those variants that are functionally more fit.
The canonical characteristics of life are an inherent capacity to:
adapt to changing environmental conditions, and
increase in complexity by multiple mechanisms, but particularly by interactions with other living organisms (including viruses).
Natural selection is the key to evolution and the main reason why Darwinian evolution persists as a characteristic of many definitions of life. Clays could never evolve an eye or a nose, or adapt behavioral strategies to exclude clays with other characteristics.
The only alternative to evolution for producing diversity would be to have environmental conditions that continuously create different lifeforms, or similar lifeforms with random and frequent “mistakes” made in the synthesis of chemical templates used for replication or metabolism. These lead to traits that gave some selective advantage in an existing community or in exploiting new habitats. This could lead to lifeforms that undergo a form of evolution without a master information macromolecule (e.g., DNA or RNA). It is difficult to imagine such lifeforms being able to “evolve” into complex structures unless other mechanisms, such as symbiosis or cell-cell fusion are available.
4.2.5. Evolution and Extraterrestrial Life#
Evolution is the key mechanism for heritable changes to occur in a population. Mutation is not the only mechanism for acquiring new genes. Lateral gene transfer appears to be one of the most important mechanisms and clearly one the earliest for creating diversity and possibly for building genomes with the requisite information to result in free-living cells (as opposed to codependent communities of “precells” that are unable to escape communal life). Lateral gene transfer is also one of the mechanisms to align genes from different sources into complex functional activities. The coevolution between two or more species is also a hallmark of evolution manifested in many ways from insect-planet interactions to the hundreds of species of bacteria involved in the nutrition of ruminant animals (e.g., cows, sheep, goats, etc.).
If the ability to undergo Darwinian evolution is a canonical trait of life no matter how different that lifeform is from Earth life, then are there properties of evolving extraterrestrial organisms that would be detectable as positive signs of life? Evolution provides an organism the opportunity to exploit new and changing environments. One piece of evidence for the probable cosmic ubiquity of evolution is that, on Earth, life occupies all available habitats and even creates new habitats as a consequence of its metabolisms.
Another hallmark of evolution is the ability of organisms to coevolve with other organisms and to form permanent (and obligatory) associations. It is highly probable that an inevitable consequence of evolution is the elimination of radically different biochemical lineages of life that may have formed during the earliest period of evolution of life. Extant Earth life is the result of either selection of the most fit lineage or homogenization of some or all fo the different lineages into a common ancestral community that developed into the present three major lineages (domains). All have a common biochemistry based on presumably the most “fit” molecular information strategies and energy yielding pathways among a potpourri of possibilities. One caveat and perhaps verification of the above statement is that some of the deeply rooted archaea exist as remnants of other lineages.
One of the apparent generalizations that can be made from extant Earth life (and the explanation of a “unity of biochemistry” in all organisms) is that lateral gene transfer is both an ancient and efficient mechanism for rapidly creating diversity and complexity. Lateral gene transfer is also an efficient mechanism for selecting the genes that are most “fit” for specific proteins and transferring them into diverse groups of organisms. The result is both the addition of new genes and the replacement of less-fit genes having a similar function. Natural selection based solely on mutation is not likely and adequate mechanism for evolving complexity.
Lateral gene transfer and endosymbiosis are probably the most obvious mechanisms for creating complex genomes that can lead to free-living cells and complex cellular communities in the short geological time available from life’s origins to the establishment of microbial communities at 3.8 Ga. An important implication of the existence of viruses or virus-like entities during the early evolution of cellular organism is that their genomes may have been the source of most genetic innovations, due to their (1) rapid replication rates, (2) high rates of mutation from replication errors, and (3) gene insertions from diverse host cells.
It is clear that both the individual organism and its community coevolve. Evolution is allowing cells to control their own evolution, where they accept or reject changes in genotypes from newly acquired foreign genes. The source of foreign genes and the kinds of genes most likely to be selected for permanence are largely not known. It is clear that the evolution of a useful trait by one organism frequently means that it is likely to be acquired by other organisms. It appears that biology is transitioning from just genes to a broader emphasis on the cell, communities, and ecosystems.
What are the limits of evolution for Earth life? This is a complex question with many different components. It involves the different biochemistries from carbon chemistry that are not found in extant Earth organisms, but could be better suited for environmental conditions that exist on other planets and moons. The technology exists to design genes and groups of genes that could lead to novel phenotypes suited to exploit new habitats and energy sources (e.g., petroleum eating bacteria). These kinds of studies would be important and perhaps essential in our quest to search for life elsewhere. Another component to the question is where we are going and what will Homo sapiens be like in 10,000 years? This is an integral part of our search for advanced extraterrestrial intelligence, which requires us to imagine our future portrait. One possible outcome will be an increasing ability to control our environment and all that is evolving based on our history in primate evolution. A more recent perspective can be found here.
4.3. Planetary Requirements for Life#
Earth offers a benign environment because it was endowed with enough volatiles to produce an ocean and a significant atmosphere. Our planet
is large enough to retain an atmosphere,
can recycle crust via plate tectonics, and
is far enough away from the asteroid belt that it is not frequently bombarded by projectiles that could cause mass extinctions.
Despite these advantages, there are still questions that astrobiologists ask for how life arises on a planet in general. Such questions are:
Does the origin of life require land masses? If land masses are required, were the small, (probably) short-lived island volcanoes sufficient for the origin of life?
Did life’s origin await the growth of larger more stable ‘continents’?
Does the origin of life require large oceans?
Does life require tidal zones to have cycling and pumping of environments that allow molecular self-assembly in lakes or ponds?
Did life actually start elsewhere and was later transplanted to Earth? Perhaps our ancestors began on Mars and traveled to Earth?
Must life ascend to land in order to develop technology?
Clearly, there is a wide variety of criteria that a planet must meet to be suitable for life, but many of these criteria are difficult to understand in detail and quantify.
4.3.1. Biogeochemical Cycles#
Carbon dioxide on our planet cycles between the atmosphere, oceans, life, fossils, other rock on a wide range of timescales. Carbonate rock (the majority of which is located in Earth’s mantle) forms the largest reservoir. Silicate rocks and atmospheric \(\rm CO_2\) can react with each other with the aid of liquid water to form silica and carbonate rocks. In some cases, living organisms are involved, while in other cases they are not.
Planets and other photosynthetic organisms remove \(\rm CO_2\) from the atmosphere, where this carbon is first stored in living organisms. Some of the carbon is returned via respiration, while a portion remains isolated for long periods of time as fossils. However, the carbonate-silicate cycle introduces the products of silicate weathering, including calcium \((\rm Ca^{++})\), bicarbonate \((\rm HCO_3^-)\), and dissolved silica \((\rm SiO_2)\), which are transported by rivers to the ocean.
Organisms (e.g., foraminifera) use these products to make shells of calcium carbonate \((\rm CaCO_3)\), while other organisms make shells out of silica. Most of these shells eventually dissolve, but some are buried in sediments on the seafloor. On a lifeless planet, calcium carbonate (i.e., chalk upon precipitation) would be incorporated in ocean sediments through nonbiological chemistry until the mineral reaches a high enough concentration to become saturated in seawater. The combination of silicate weathering plus carbonate precipitation is given in Eqn. (3.76). Thermal processing of buried carbonate rocks returns \(\rm CO_2\) to the atmosphere.
The episodic return of \(\rm CO_2\) to the atmosphere affects the climate, where for the past few million years, the climate cycle on Earth has alternated between ice ages and interglacials (i.e., periods between ice ages). When the climate cools, ice sheets form because the ice is highly reflective (i.e., has a high albedo) and this change leads to further cooling through a positive feedback loop.
Evidence suggests that most (or all) of Earth’s surface was covered by ice about \(700\ {\rm Myr}\) ago in a state known as snowball Earth. Significant ice ages (like snowball Earth) may have precipitated large scale changes to the biosphere, where an ice age appears to correlate with both the great oxidation event and the Cambrian explosion. Positive feedbacks can have large effects on habitability, even with small changes in input parameters.

Fig. 4.6 Illustration of the long-term (geological) carbon cycle fluxes. Shown are the long-term fluxes (in petagrams of carbon/yr) in the GENIE (Grid ENabled Integrated Earth system) model at steady state. In red are sources of \(\rm CO_2\) to the atmosphere or ocean, and in dark blue are sinks of \(\rm CO_2\). Figure Credit: Colbourn, Ridgwell, & Lenton (2015).#
However, variations of parameters can damp the atmospheric \(\rm CO_2\) abundance, which results in a negative feedback loop. This process buffers the terrestrial climate on timescales of \(100\ {\rm Myr}\). Carbon dioxide is an important greenhouse gas, where the reaction rate of \(\rm CO_2\) with silicate rocks can increase exponentially with temperature. But the other phases of the \(\rm CO_2\) cycle are virtually temperature independent. If it gets hot, weathering rates increase and the \(\rm CO_2\) abundance drops. During colder times, the reverse occurs and \(\rm CO_2\) increases. Variations in the atmospheric abundance of \(\rm CO_2\) are thought to have played a major role in maintaining moderate climates on Earth for most of our planet’s history (see Fig. 4.6 for carbon flux rates).
The carbon cycle also limits the abundance of atmospheric \(\rm CO_2\) on billion-year timescales. Ocean basalts remove \(\rm CO_2\) from seawater at a rate that increases with the \(\rm CO_2\) abundance in seawater, which varies in sync with its atmospheric abundance. These carbonates descend into the Earth’s mantle as plates are subducted. Heating within the Earth’s mantle frees the \(\rm CO_2\), which is subsequently returned to the atmosphere via volcanic activity. This process does not depend on climate and acts to buffer the atmospheric \(\rm CO_2\) abundance rather than climate variations.
Nitrogen is often a limiting nutrient for life on Earth, despite abundant atmospheric \(\rm N_2\). Nitrogen fixation is the process by which nitrogen is taken from its relatively inert molecular form in the atmosphere and converted into soluble nitrogen compounds, such as ammonia, nitrate, and nitrogen dioxide (see Fig. 4.7). Few organisms can metabolize nitrogen unless it has been fixed from the atmosphere.
In the anoxic (oxygen poor) prebiotic atmosphere of the young Earth, lightning oxidized \(\rm N_2\) via the reaction
The nitric oxide \((\rm NO)\) was then converted to nitrosyl hydride \((\rm HNO)\), which is soluble in water. Lightning thereby provided a modest flux of fixed nitrogen, which may have been important to early life. In an atmosphere containing some methane \((\rm CH_4)\), nitrogen fixation can progress via \(\rm HCN\), which is hydrolyzed in solution to form ammonium \((\rm NH_4^+)\).
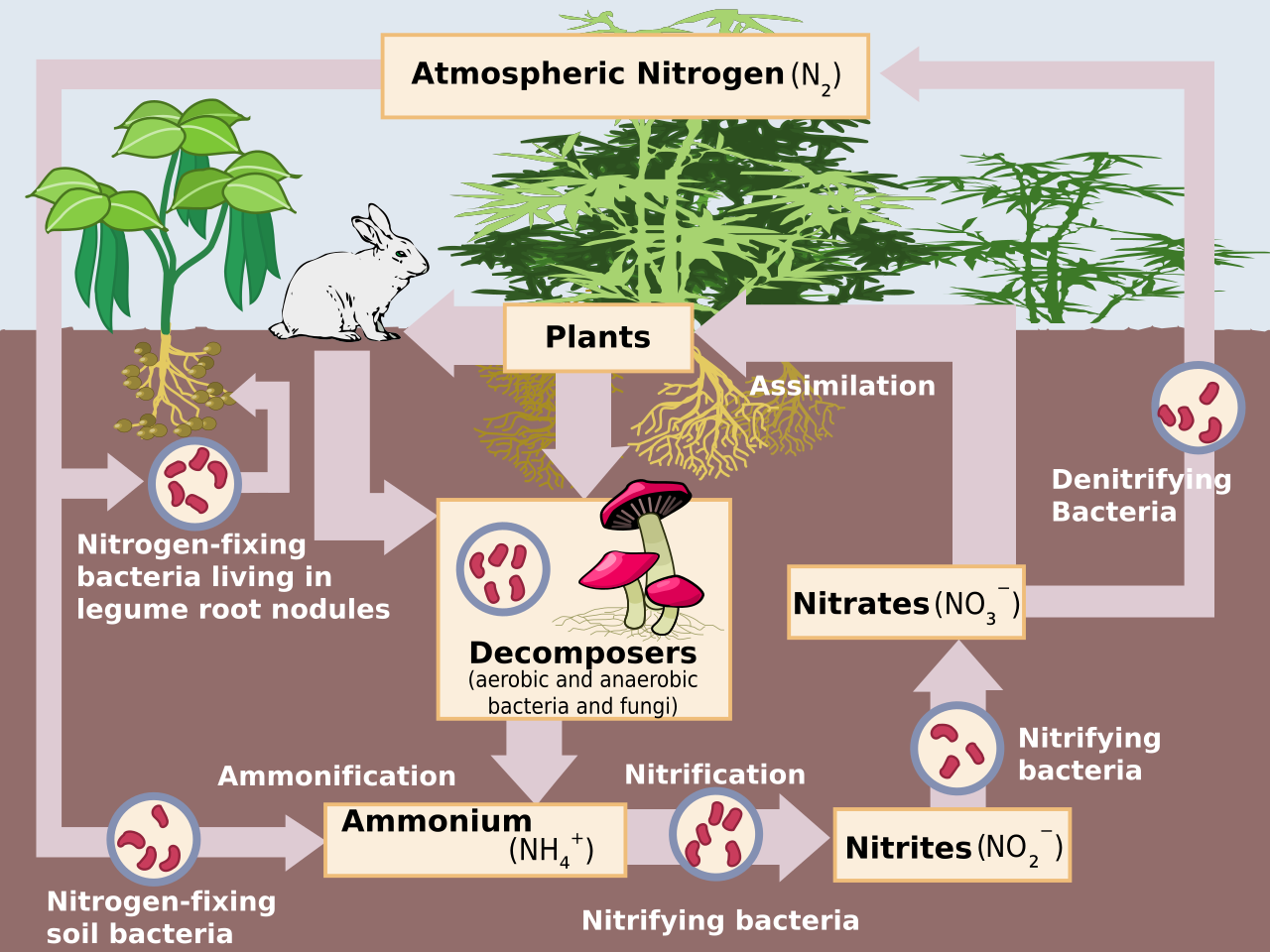
Fig. 4.7 Diagram illustrating the nitrogen cycle, where atmospheric nitrogen enters ecosystems through fixation. Image Credit: Wikipedia:Nitrification.#
Biological fixation of nitrogen arose when fixed \(\rm N\) became scarce, which may have been prior to the last common ancestor of present-day Earth life, and is strictly an anaerobic process. Biological fixation of nitrogen occurs when anaerobes developed the capability of nitrogen fixation, and \(\rm NH_4^+\) probably became the dominant form of combined nitrogen in Earth’s oceans. Once our planet’s atmosphere contained significant quantities of \(\rm O_2\), then ammonium ions were oxidized to nitrite \((\rm NO_2^-)\) and then converted to nitrate \((\rm NO_3^-)\) by bacteria nitrification. The reverse process (nitrates back to molecular nitrogen) is called denitrification.
Note
The term anoxic is used to describe environments without molecular oxygen, while anaerobic refers to organisms that are able to survive without molecular oxygen.
4.3.2. Gravitational and Magnetic Fields#
Biomechanics depends on material strength, gravity, and other physical properties related to viscosity, which means that organisms do not scale simply scale with size.
Larger animals require more support, so elephants need to have more bulky legs than a scaled-up mouse.
Birds use a fundamentally different mechanism to fly than do insects.
Single-celled organisms have little inertia (compared with viscous drag) moving in liquid water, so they use different locomotion strategies than do larger creatures.
These factors must be accounted when considering planets with a different surface gravity than Earth. Planetary gravity is also important through its effect on escape of atmospheric gases and mountain building.
Earth has a much larger magnetic field than do any of the other terrestrial planets within our Solar System. Are magnetic fields necessary for life? Direct effects of the magnetic field are minor, where a few species are known to use them as aids for navigation (but most organisms do not). Earth’s magnetic field also plays a protective role by stopping charged particles from eroding the atmosphere or reaching the surface.
Such particles could have profound direct and indirect effects on life, but they are unlikely to sterilize a planet that has a sufficiently massive atmospheric blanket. During magnetic field reversals, the dipole component of Earth’s magnetic field drops to near zero and yet life persists. A subsurface biosphere would be weakly affected by charged particles hitting the planet’s surface and thus, does not require the protection of either a planetary magnetic field or an atmosphere.
4.3.3. Can Moonless Planets Host Life?#
The moon’s orbit correlates with many cycles on Earth, where some species of sea turtles lay their eggs on beaches only at full Moon. The nocturnal behavior of various species requires the light of the Moon, and clearly different ecological niches would be available on a moonless planet. Although these factors would affect some aspects of life significantly, they cannot be regarded being necessary (i.e., without a large moon, there is no Earth life) nor sufficient (i.e., there is a large moon, therefore there must be life) for life to exist. However, other effects of the Moon have been suggested to be more essential to life on Earth.
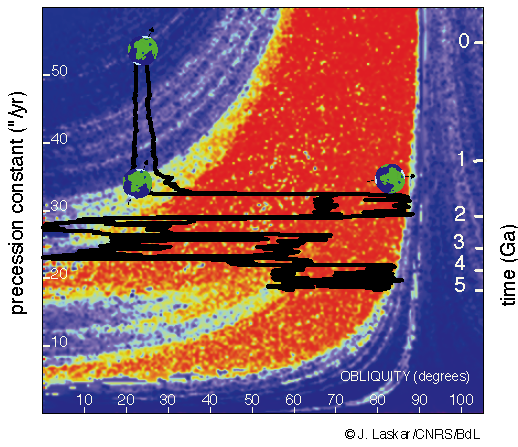
Fig. 4.8 Diagram illustrating possible changes in obliquity (color-coded) for a moonless Earth. The present-day Earth (with the Moon’s effects included) has an obliquity of \(23.3^\circ\) and spin precession constant of \({\sim}51\ {\rm ^{\prime\prime}/yr}\), which results in a small obliquity variation (blue). A moonless Earth (with a precession constant \({\sim}15-30\ {\rm ^{\prime\prime}/yr}\)) would have a much larger obliquity variation (up to \({\sim}60^\circ\); in red). Image Credit: Jacques Laskar from Laskar, Joutel, & Robutel (1993).#
The Moon stabilizes Earth’s obliquity (see Fig. 4.8) and thereby its climate (see Fig. 3.23). In contrast, perturbations from the other planets produce substantial variations in the martian obliquity, which probably are responsible for the patterns observed in Mars’ layered polar terrains. However, planets with
retrograde spins,
more rapid spin rates, or
those in planetary systems with a different orbital architecture from our own
can have stable obliquities, even without a moon (e.g., Lissauer, Barnes, & Chambers (2012); Barnes et al. (2016); Quarles, Li, & Lissauer (2019); Quarles et al. 2020). The climate of a planet with more ocean and less continental mass than Earth would be less sensitive on planetary obliquity.
The Moon is the primary body responsible for ocean tides on Earth (in the present era), and it produced substantially larger tides when it was closer to Earth billions of years ago. Tidal zones at the boundaries between sea and land on Earth are subject to cyclic variations in conditions with alternating wet and damp/dry periods. Very productive ecosystems currently exist within tidal zones. organic molecules can be concentrated by evaporation in tide pools and be supplied with repeated addition of nutrients. Life on Earth many have originated in tidal regions, where a large moon is advantageous to the formation of life. Since solar tides are negligible, a moon cannot be viewed as essential to life on this account.
The leading model for the Moon’s formation is the giant impact theory. This large impact removed volatile elements and compounds from Earth, where such a devolatilization may have been required for Earth itself to become suitable for life. The video below (from Miki Nakajima) shows the standard giant impact model (Hartmann and Davis 1975; Cameron and Ward 1976). Models of planetary growth suggest that most terrestrial volatiles come from a small fraction of the material composing our planet, so an otherwise Earth-like planet could simply accrete fewer volatile compounds than Earth. Alternatively, a planet could be devolatilized by a nearly head-on, mega-impact that would not loft enough material into orbit to produce a large moon.
4.3.4. Giant Planets and Life#
Giant planets are unlikely abodes for life because any solid surface that they might have would be at extremely high pressure. Living organism occupy essentially all known environments on or below the surface of our planet in which liquid water is present.
Despite billions of year of evolution, no known terrestrial organisms have adapted to a purely aerial life cycle. Although, Sagan & Salpeter (1976) hypothesized how an aerial ecosystem could develop on a gas giant. Even though moderate temperate zones occur within giant planet atmospheres, parcels of gas do not remain in place, but are repeatedly mixed downwards by convection into regions that are too hot for organic molecules to survive.
Giant planets may harbor habitable moons, where moons of giant planets seem to have a similar potential for habitability as do terrestrial planets of the same mass and distance from their star. Formation circumstances may result in different average compositions, but the distributions of composition os such similarly sized moons and planets probably overlap.
Tidal interactions between large moons and giant planets are likely to be much more substantial than between stars and planets within the respective habitable zones due to the close proximity of moons to their host planets. This implies that such moons are probably in synchronous orbits. The consequences of such a spin-orbit resonance would be far less than for planets in synchronous rotation within the habitable zones of faint, low-mass, M-dwarf stars.
The length of the day on such a moon would be slowed to approximately the moon’s orbital period about its planet. If it was in orbital resonance with another moon, energy from their coupled tidal recession from the planet would be deposited in one or both moons. This energy source could produce subsurface oceans, as is likely the case on Jupiter’s moon Europa.
Giant planets can also affect the habitability of a terrestrial planet orbiting the same star, where they can destabilize the terrestrial planet’s orbit causing an increase in the terrestrial planet’s eccentricity. The increased eccentricity allows for the planet to collide with another nearby planet or to ejection into interstellar space with a close approach to the host star.
Giant planets can cause obliquity variations that affect the climate of terrestrial planets (as would be the case for a moonless Earth). Giant planets affect the flux of impactors that bombard terrestrial planets. Such impacts can have a devastating effect on life (as was the case for the dinosaurs).
Giant planets may be important during the formation of habitable planets. It is likely that giant, vulcan planets migrated through the habitable zone on their way to the final orbit that is very close to their host star. As a result, they may have cleared material from that region to prevent the formation of terrestrial planets large enough to retain an atmosphere.
Giant planets that orbit farther from their star may have positive effects on terrestrial planet habitability, where the small fraction of volatiles possessed by Earth were probably diverted from colder regions of the protoplanetary disk by perturbations from Jupiter and Saturn.
4.4. How Life Affects Planets#
Life an the environments where it flourishes on Earth have been closely intertwined for billions of years. The first microbes consumed the most readily available food, and subsequent life had to develop new energy sources to survive. The most productive energy source developed was to harness solar energy via photosynthesis, where the most efficient form of photosynthesis releases oxygen \((\rm O_2)\) as a byproduct.
Oxygenic photosynthesis also provides a significant sink for atmospheric carbon dioxide. Microbes living in the digestive systems of cattle excrete methane into the atmosphere. Large forest fires can fill the atmosphere with soot, warming the atmosphere but shielding the surface from solar radiation. The burning of extant and extinct biomass releases gases and particulates into the environment.
The presences of life has profound effects on the surface morphology of Earth. Forests cover large fractions of continental crust, which changes the
albedo (including seasonal variations),
soil composition, and
the local climate.
Microorganisms also change the soil composition through metabolism. The roots of land plants stabilize soil and reduce erosion, while beavers dam rivers. Humankind has large effects on the surface morphology through building and mining projects, pavement that affects drainage, and altering the composition of the atmosphere.
The second most abundant gas in Earth’s present-day atmosphere is oxygen \((\rm O_2)\), where this has not always been the case (see Fig. 3.25). Many gaps exist within the geologic record, but it is clear that the atmosphere of the early Earth was reducing. The oxygenation of Earth’s atmosphere occurred over billions of years, with an especially significant increase \({\sim}2.2\ {\rm Gyr}\) ago.
Oxygenic photosynthetic bacteria produce \(\rm O_2\) as a waste product, while methanogenic organisms release \(\rm CH_4\). Some of this methane rises to the stratosphere and is photodissociated. A portion of the hydrogen formed by dissociation continues moving upwards to the exosphere and subsequently escapes. For the first few billion years, Earth’s atmosphere and crust were sufficiently reducing that they were capable of absorbing the released oxygen. Some processes (e.g., hydrogen escape and long-term sequestration of carbon within the crust and the mantle) diminished the environment’s ability to absorb oxygen, and eventually the \(\rm O_2\) abundance in our planet’s atmosphere increased substantially.
Biological processes play an important role in the carbon cycle. Today’s fossil fuels (e.g., coal, crude oil, and ‘natural gas’ (methane)) were produced from partially decayed planets and many now extinct organisms. Shells of some marine organisms sequester carbon and other elements for long timescales. The simultaneous long-term presence of abundant mutually reactive gases (e.g., oxygen and methane) in a planet’s atmosphere requires a highly non-equilibrium process, such as oxygenic photosynthesis.
Life has persisted on Earth for well over three billion years despite a significant increase in the Sun’s luminosity, a large reduction of the amount of escaping geothermal heat, and many other changing conditions on our planet. This endurance suggests that life itself may play a role in regulating the environment to allow for its continued existence. Figure 4.9 illustrates many of the factors that affect a planet’s habitability in which the planet’s biology plays a part of a much larger web.
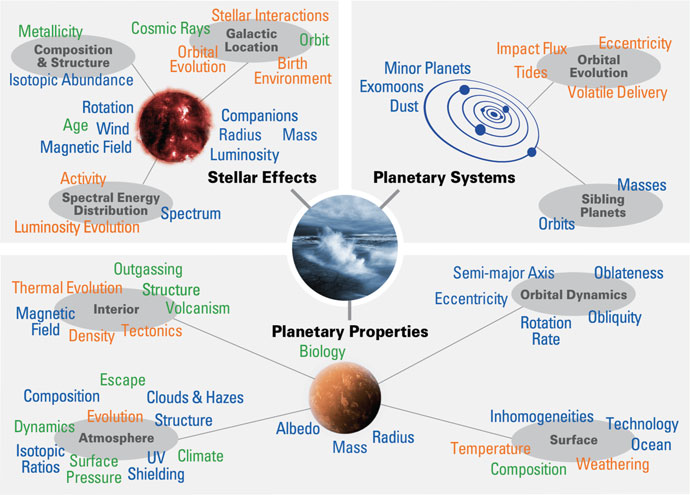
Fig. 4.9 Factors affecting habitability. This diagram shows currently understood planetary, stellar, and planetary system properties that may impact planetary habitability. Figure Credit: Meadows & Barnes (2018).#
4.5. Homework#
Problem 1
Summarize the attempts to define life and contrast the differences between the four main definitions. Which definition for life is the most popular and why?
Problem 2
What are the parts of a definition? How does a definition guide (bias) astrobiologists in the search for life elsewhere?
Problem 3
There are some ecological limits to life. Summarize these limits and explain how they can be used to make inferences for the potential for life in the Solar System.
Problem 4
Describe the process of evolution as envisioned by Darwin. How does it proceed at the molecular level?
Problem 5
There are three primary mechanisms for organisms to acquire new genes. Summarize these mechanisms and propose what environmental conditions that would best suit each mechanism.
Problem 6
Are there any alternatives for life to gain complexity without Darwinian evolution? Summarize at least one of the alternative hypotheses.
Problem 7
Draw diagrams illustrating main features of the Carbon and Nitrogen cycles. Explain how these cycles help support Earth-like ecosystems.
Problem 8
Describe how external factors (e.g., Magnetic fields, large moons, and giant planets) may affect the potential for life to arise on an Earth-like planet.